DOI:
10.1039/C0NR00547A
(Paper)
Nanoscale, 2011,
3, 303-308
Functionalization of surfactant wrapped graphene nanosheets with alkylazides for enhanced dispersibility†
Received
29th July 2010
, Accepted 13th September 2010
First published on 4th November 2010
Introduction
Graphene is an infinite 2D sheet of sp2 bonded carbon atoms with extended electronic conjugation. Since its discovery in 2004, the material has triggered enormous interest in both fundamental and applied science communities because of the unique electrical, thermal and mechanical properties.1–8 However, use of this new material in electronic devices, sensors and photovoltaic devices has been hindered by the unavailability of stable and processable graphene structures.9–13 Recently, many methods have been developed for the bulk production of graphene sheets. One of the commonly used method is mechanical cleavage of highly ordered pyrolytic graphite (HOPG) using Scotch Tape.2 The sheets obtained in this manner may contain different numbers of graphene layers and can be easily removed by solvent treatment or sonication.14 Another strategy relies on the use of graphite oxide (GO). GO is hydrophilic owing to the presence of hydroxyl and epoxide functional groups in the basal plane. Chemical modification of GO in the presence of various stabilizers such as amphiphilic polymers,15pluronic co-polymers,16hydrazine,17pyrene derivatives,18phenyl isocyanates,19octadecylamine,20 and diazonium salts21 has been reported to improve its processability. However, the strong tendency of these layers to stack limits its potential applications.22Sonication assisted dispersion of graphene in N,N-dimethyl formamide (DMF)23 or N-methyl pyrrolidone (NMP)24 and electrochemical methods25 have also been reported, however only small graphene fragments can be obtained through this method.
Given the interest in graphene chemistry, it is desired to have convenient dispersion routes to increase the availability and processability of graphene. To improve the solution processability of graphene, a few methods that have been employed for the functionalization and stabilization of carbon nanotubes (CNTs) can be adopted.26,27 Moreover, chemical functionalization of the carbon network in graphene by grafting atoms or molecules is very important because this provides a method for the introduction of interesting photoactive molecules for the development of optoelectronic materials. An interesting functionalization to try on graphene is azide addition because many organic azides have been used for the covalent modification of single walled and multi walled CNTs.28
Based on this concept, a mild covalent functionalization of cetyltrimethylammonium bromide (CTAB) wrapped graphene sheets using a series of azides, which include alkylazides (hexyl and dodecyl), 11-azidoundecanol (AUO), and 11-azidoundecanoic acid (AUA), is developed. Besides the azido group, other functional groups such as hydroxyl and carboxylic groups are incorporated to enhance the dispersibility in common organic solvents. The functionalized sheets are characterized by Fourier transform infrared spectroscopy (FTIR), Raman spectroscopy and various imaging techniques. In addition, the reactive sites on the graphene sheets were marked using gold nanoparticles. Free carboxylic acid groups present in the AUA functionalized sheets bind with gold nanoparticles, indicating that the reaction has taken place not just at the edges but also at the internal C
C bonds of graphene. In short, our results show that the covalent functionalization using azides provides a useful platform for the synthesis of functional graphene nanocomposites using gold nanoparticles.
Results and discussion
Characterization of the functionalized graphene sheets
Scheme 1 illustrates the process of the covalent functionalization of CTAB stabilized graphene sheets with various alkylazides. CTAB assisted exfoliation of graphite is reported previously (Fig. S1 of the ESI†).29 Nitrenes of the azides are inserted into the double bonds on graphene sheets and the alkyl chains with different functional groups at the terminals. It is conceivable that the presence of long alkyl chains and polar functional groups improves the dispersibility. The functionalization with dodecylazide, AUO and AUA improved the dispersibility of graphene sheets in common organic solvents, such as toluene and acetone, and the dispersions were stable for a few days. In the case of hexylazide, the dispersions were not stable and the sheets were agglomerated (Fig. S2 of the ESI†). It can be attributed to the shorter alkyl chain of the hexylazide. In the case of AUO and AUA, the functionalization led to enhanced dispersibility in common solvents.
 |
| Scheme 1 Schematic representation of the covalent functionalization of graphene sheets with various alkylazides. | |
The presence of polar functional groups on the graphene surface is desirable because it assists in enhancing the dispersibility. Besides, functional groups such as –COOH and –OH can anchor metal nanoparticles onto the surface, which may help in visualizing the reactive sites. Hence, gold nanoparticle synthesis was attempted in the presence of both AUO and AUA functionalized graphene sheets. In the presence of AUO functionalized sheets, the gold nanoparticles were aggregated on their surface. Transmission electron microscopic (TEM) images of the AUO functionalized sheets and the gold nanocomposites are given in Fig. S3 of the ESI.† A possible reason for this may be the poor stabilizing/coordinating effect of hydroxyl groups. However, gold nanocomposites with AUA functionalized graphene sheets were found to be stable without any visible aggregation. Hence, we focused our studies on AUA functionalized graphene owing to the fact that the presence of –COOH groups on the surface might enhance the metal nanoparticle-graphene composite stabilities and dispersibility. The dispersibility of the resultant product was poor when a 1
:
1 weight ratio of graphene to AUA was used for functionalization. It could be due to minimal functionalization on the graphene sheets. The TEM image of the dispersed sample is given in Fig. S4 of the ESI.† Treatment of graphene with AUA at a 1
:
10 ratio led to the formation of a solid considerably dispersible in common organic solvents. These sheets were found to be easily dispersible in polar solvents with a mild sonication. These samples were used for subsequent experiments. The dispersibility was found to be high in DMF. In the case of CTAB stabilized graphene sheets, the maximum dispersibility achieved was ∼0.005–0.01 mg mL−1, but for AUA functionalized sheets, it could reach up to a maximum of ∼0.05–0.1 mg mL−1.
Fig. 1a and 1b show the TEM micrographs of the CTAB stabilized and AUA functionalized graphene sheets, respectively. Due to the distortions caused by the covalent functionalization, sheets attain a crumpled topology (Fig. 1b). As reported previously, crumpling and scrolling are intrinsic properties of thin graphene sheets, which is due to the extra thermodynamic stability of the 2D membranes arising from microscopic crumpling, either by bending or buckling.30
To verify the covalent functionalization of graphene sheets with AUA, FTIR was performed (Fig. 1c). Traces A and B correspond to the IR spectrum of AUA and AUA functionalized graphene sheets (1
:
10 w/w of graphene to AUA), respectively. The peak at ∼2100 cm−1 corresponds to the azido stretching31 and that at 1730 cm−1 to the carbonyl stretching from the –COOH group. Absence of an azido stretching peak in AUA functionalized graphene sheets implies covalent functionalization.28bAzides are added onto C
C bonds on graphene with the elimination of N2. The IR spectrum of the AUA functionalized graphene sample showed the presence of C–N (∼1128 cm−1) and C
C stretching (∼1630 cm−1).
Fig. 1d shows the Raman spectra of CTAB stabilized graphene sheets (trace 1) and the AUA functionalized sheets with 1
:
1 and 1
:
10 w/w of graphene to AUA (traces 2 and 3, respectively). The peak centered at ∼1350 cm−1 corresponds to the disorder induced D band and that at ∼1580 cm−1 to the E2g phonon of sp2 atoms.32 The intensity of the D band is directly proportional to the amount of disorder present in the sheets. The D band intensity increased considerably with azide functionalization, owing to the disorders induced by the addition of nitrenes to the double bonds. Another prominent feature in the Raman spectra of graphene samples is the 2D (or G′) peak. No defects are required for the existence of this band and it is seen even when no D band is present.24 The number of layers present in the graphene sheets can be evaluated from the shape of 2D peak.33Graphene sheets with less than five layers have very broad 2D peaks, which can be clearly seen in the case of both CTAB stabilized and AUA functionalized graphene samples.
The thickness and morphology of the functionalized graphene sheets was evaluated using atomic force microscopy (AFM) (Fig. 2a). From the cross section analysis of one of the flakes, the thickness was found to be 1.12 nm (Fig. 2b). Analysis of a large number of AFM images revealed graphene flakes with lateral dimensions of 500–2000 nm and thicknesses in the range of 1–1.3 nm (statistical analysis on the thickness is given in Fig. S5 of the ESI†).
 |
| Fig. 2
AFM images of (a) the AUA functionalized graphene sheets and (b) the section analysis of one of the flakes. | |
The thickness of a monolayer of graphene sheets is 0.34 nm as predicted by theory,3 while our samples showed an average thickness of 1.3 nm, indicating the presence of 2–3 layered graphene sheets. A slight increase in the thickness of the sheets as compared with the CTAB stabilized sheets29 can be attributed to the presence of long alkyl chains present on both sides of the sheets.
Finally, scanning tunneling microscopy (STM) analysis was carried out on the samples to detect the disorders caused by functionalization. A highly ordered hexagonal lattice of graphene was seen in the case of CTAB stabilized graphene flakes (Fig. 3a). Small nonuniformities arose from the presence of loosely bound residual surfactants. However, the STM image of AUA functionalized sheets clearly showed many bright regions, lacking the hexagonally ordered arrangement. These disorders could be due to the functionalization on certain areas (Fig. 3b). The functionalized regions are highlighted with white contours.
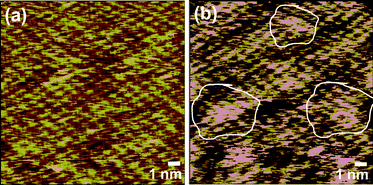 |
| Fig. 3
STM images of (a) CTAB stabilized and (b) AUA functionalized graphene sheets. | |
Gold-graphene nanocomposites
It is conceivable that the presence of –COOH groups present in the anchored AUA moieties on graphene helps in binding the nanoparticles on the graphene surface. Hence, gold nanoparticles were synthesized in the presence of AUA functionalized graphene sheets. Fig. 4a shows the absorption spectra recorded for the AUA functionalized graphene sheets (trace A) and the gold-graphene nanocomposite solutions (trace B), respectively. The inset of Fig. 4a shows the photograph of the corresponding solutions (solutions are diluted 10 times for better contrast). The gold-AUA functionalized graphene nanocomposites formed were stable for several days, with no visible aggregation. It is well known that surface plasmon resonance (SPR) of the metal nanoparticles depends on their size.34 In our sample, the SPR band was around 520 nm, which corresponds to a size >5 nm. The size and shape of gold nanoparticles on graphene sheets were further confirmed using TEM and AFM measurements.
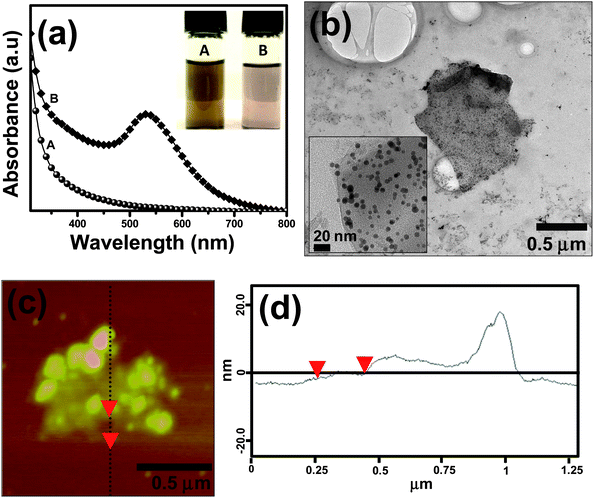 |
| Fig. 4 (a) UV-vis spectrum of the AUA functionalized graphene sheet (trace A) and that of the gold-graphene nanocomposite (trace B) solutions. Inset of Fig. 4a shows the photograph of (A) functionalized graphene solution and that of (B) the gold-AUA graphene composite in DMF. (b) TEM image and (c) the AFM image with (d) the cross sectional analysis of the gold-graphene nanocomposite sheets. | |
Homogeneous grafting of gold nanoparticles on the graphene film is visible in the TEM image (Fig. 4b).
From the TEM images, the average size of the nanoparticles was found to be 9.5 ± 2.2 nm. The AFM image also depicts fine distribution of nanoparticles on the graphene nanosheets (Fig. 4c). From the cross sectional analysis (Fig. 4d), the height from background was found to be ∼1.26 nm, corresponding to the AUA functionalized graphene sheet thickness. The smaller peaks seen in the cross section were from the gold nanoparticles anchored on the graphene flakes. Even though the reaction mechanism is not clear at this stage, it is believed that the nanoparticles are adhered onto the functionalized graphene sheets via electrostatic interactions and –COOH groups.
The uniform distribution of the nanoparticles on the AUA functionalized graphene indicates that the reaction has taken place not just at the edges of the graphene sheets but also at the central C
C bonds. This is very interesting because this method of functionalization can open up new reaction routes for the synthesis of graphene based functional composite materials.
Primary investigations on the applicability of the graphene-gold nanoparticle composite as a conducting composite material were carried out using I–V measurements. Fig. 5 shows the I–V plots of drop-casted films of AUA functionalized graphene sheets and the gold-graphene nanocomposite. It is evident from the graph that AUA functionalized graphene sheets show low conductivity due to the lack of extended π-conjugation. The film showed a conductance of 22 nA at an applied voltage of 10 V (trace A). In the case of gold-graphene nanocomposites, the conductivity increased approximately three fold as compared to pure functionalized sheets. Gold-graphene nanocomposite films showed a conductance of 76 nA at an applied voltage of 10 V (trace B). The increase in conductance for the gold-graphene nanocomposites can be attributed to the presence of attached gold nanoparticles. Both the drop-casted samples contained the same concentration of graphene sheets. Hence, it can be concluded that the increased conductivity is due to the embedded gold nanoparticles. Some of the earlier works on GO based composites showed 6 fold35 and 270
000 fold36,37 increases in conductance on reduction of GO by chemical means. This increase can be attributed to the restoration of π–π electronic conjugation or presence of metallic impurities on the graphene surface. In our case, there is no restoration of the extended conjugation or use of metallic reducing agents. Therefore, the increase in conductivity is mainly due to the embedded Au nanoparticles, which create the conducting pathways. The conductivity obtained is lower than that of bulk graphite since the measurement was done on drop-casted films with multiple discontinuities. At the present stage, it will be difficult to comment on the mechanism of electrical conduction, despite the great potential of such materials. Attempts to anchor other metal nanoparticles such as Pt and Fe onto the functionalized graphene sheets and their electrical property investigations are currently underway in our lab.
 |
| Fig. 5
I–V plots of films of (A) AUA functionalized graphene sheets and (B) gold-graphene nanocomposites. | |
Conclusions
In conclusion, we have demonstrated a mild and simple functionalization technique for dispersing graphene sheets. Surfactant wrapped sheets were functionalized with various alkylazides. Sheets functionalized with azides with longer alkyl chains and polar functional groups showed enhanced dispersibility in common organic solvents such as acetone and toluene. AUA functionalized sheets showed better dispersibility and stability in the solution state. The functionalizations were confirmed using various imaging and spectroscopic techniques. The thicknesses of the functionalized sheets were found to be in the range of 1–1.3 nm. This approach can be used for the introduction of various functional moieties into graphene sheets, and this may prove to be very useful for applications in electronic devices, and graphene based composite materials. Anchoring of gold nanoparticles onto AUA functionalized graphene sheets were also demonstrated. This gold-graphene nanocomposite was stable in solution for several days and showed threefold increase in conductivity as compared to the pure AUA functionalized sheets. Such metal nanocomposites could be promising candidates for catalytic and electronic applications.
Experimental
Materials
Sodium azide (NaN3), 11-bromoundecanoic acid, 11-bromo-1-undecanol, dodecyl bromide, hexyl bromide, anhydrous sodium sulfate (Na2SO4), CTAB, graphite powder (> 45 μm), chloroauric acid (HAuCl4·3H2O) and sodium borohydride (NaBH4) were obtained from Sigma Aldrich and used as received. The solvents were of analytical grade and were obtained from local suppliers.
Analysis and instruments
NMR spectra of the synthesized organic compounds were recorded using a Bruker ACF spectrometer operating at 300 MHz. Chloroform-d (CDCl3) was used as the solvent and tetramethylsilane (Me4Si) as internal standard. The electron impact mass spectrum was recorded using a Finnigan MAT95XL-T mass spectrometer. The UV-visible spectrum was recorded using a Shimadzu 1601 PC spectrophotometer. FTIR spectra were recorded at 1 cm−1 resolution in the wave number range of 4000–400 cm−1 using a Bio-Rad FTS 165 FTIR spectrophotometer. High resolution transmission electron micrographs (HRTEM) were taken using a JEOL JEM 2010 F electron microscope, operating at 200 keV. A dilute suspension of the graphene flakes drop-casted on the copper grid was used for HRTEM measurements. STM measurements were performed on Au(111) substrate (Goodfellow, UK) using a NanoScope IIIa MultiMode SPM (Digital Instrument, Veeco Metrology Group) with electrochemically etched Pt(.8) Ir(.2) tips (0.25 mm diameter). A drop of graphene solution deposited on Au(111) substrate was used for the STM measurement. STM images were obtained at a tunneling current of 2 pA and a bias voltage of 50 mV. AFM images were recorded using an Agilent AFM with Pico plus molecular imaging system in the non-contact mode. Samples for AFM were prepared by spin-coating a dilute suspension of graphene on freshly cleaved mica at a speed of 5000 rpm and then dried at room temperature. Raman spectra were recorded with a Renishaw system-2000 operating at an excitation wavelength of 532 nm. A source meter (Keithley 6430) was used for the current–voltage (I–V) measurements, at room temperature. The samples for electrical characterization were prepared by drop-casting the DMF suspensions of the samples on a Si substrate with 300 nm SiO2 coating. The graphene flakes were found to be intercalated and formed a continuous network over the SiO2 surface. Tungsten probes with a tip diameter of 2.4 μm were precisely positioned over the graphene flakes at a distance of 10 μm.
Preparation of CTAB stabilized graphene sheets29
In brief, 100 mg of graphite powder were sonicated with CTAB solution in glacial acetic acid for 4 h. The resultant solution was then refluxed for 48 h under nitrogen atmosphere. The reaction mixture was left to stand overnight to allow the unstabilized flakes to settle down. The supernatant was decanted and centrifuged at 20
000 rpm for 45 min. The resultant black residue obtained could be re-suspended in DMF.
Synthesis of 11-azidoundecanoic acid (AUA)38
NaN3 (294 mg, 4.52 mmol) was dissolved in a solution of 11-bromoundecanoic acid (1 g, 3.77 mmol) in dimethyl sulfoxide (DMSO) (20 mL). It was stirred at room temperature overnight. The reaction mixture was diluted with water and 1 M HCl was added. The aqueous phase was extracted (3 × 15 mL) with ethyl acetate (EtOAc) and the combined organic layers were washed with water, dried over anhydrous Na2SO4 and evaporated to get the 11-azidoundecanoic acid (0.67 g, 78%) as a pale brown oil (elemental analysis, found: C, 58.2; H, 9.2; N, 18.5; O, 14.1. C11H21N3O2 requires C, 58.12; H, 9.32; N, 18.49; O, 14.08%); δH(300 MHz; CDCl3; Me4Si) 10.25 (1 H, s, COOH), 3.22 (2 H, t, CH2N3), 2.31 (2 H, t, CH2COOH), 1.59–1.54 (4 H, m, CH2CH2N3 and CH2CH2COOH) and 1.27 (12 H, m, 6 × CH2); m/z (EI) 140.1 (M+ − C4H7O2C7H14N3 requires 140.12), 126 (5%), 112 (8), 98 (9), 84 (26), and 70 (100). Detailed synthesis and characterization of all the other azides are given in the supporting information.†
Functionalization of CTAB stabilized graphene sheets with 11-azidoundecanoic acid (AUA)
For AUA functionalized graphene sheets, two different weight ratios of graphene to AUA (1
:
1 and 1
:
10) were used to check the changes in dispersibility with the extent of functionalization. The weight of graphene was kept constant for convenience. Briefly, 20 mg of the CTAB assisted exfoliated graphene29 was dispersed in 50 mL of O-dichlorobenzene (ODCB). The solution was purged with nitrogen and preheated to 160 °C. Different amounts of AUA (20 mg and 200 mg, respectively) in 5 mL of ODCB were then added drop-wise to the reaction mixture over a period of 20–30 min. The temperature was maintained at 160 °C for 45 min, after which the product was cooled to room temperature. The suspension was diluted with acetone to flocculate the functionalized graphene sheets. Subsequent washing and centrifugation resulted in the removal of all by-products. The graphene sheets were decanted and dried.
Preparation of gold-graphene nanocomposites
Gold nanoparticles were synthesized in the presence of AUA functionalized graphene sheets. To 10 mL of the functionalized graphene suspension (∼ 0.05 mg mL−1) in DMF, HAuCl4 solution was added so that the final concentration of Au3+ was 10 mM. It was then reduced with the addition of 500 μL freshly prepared NaBH4 (2 × 10−4 M) solution with continuous stirring. The color of the solution gradually changed from grayish black to dark pink. Formation of nanoparticles was confirmed using spectroscopic and microscopic techniques.
Acknowledgements
The authors thank National Research Foundation (NRF), Singapore for research funding, NUS Nanoscience and Nanotechnology Initiative (NUSNNI) for graduate scholarship, and the Department of Chemistry, National University of Singapore (NUS) for technical support. Dr Yongping Zhang (Singapore Millennium Foundation Postdoctoral Fellow, NUS) is acknowledged for helping with the STM measurements.
Notes and references
- N. A. Kotov, Nature, 2006, 442, 254–255 CrossRef CAS.
- K. S. Novoselov, A. K. Geim, S. V. Morozov, D. Jiang, Y. Zhang, S. V. Dubonos, I. V. Grigorieva and A. A. Firsov, Science, 2004, 306, 666–669 CrossRef CAS.
- K. S. Novoselov, D. Jiang, F. Schedin, T. J. Booth, V. V. Khotkevich, S. V. Morozov and A. K. Geim, Proc. Natl. Acad. Sci. U. S. A., 2005, 102, 10451–10453 CrossRef CAS.
- J. S. Bunch, A. M. van der Zande, S. S. Verbridge, I. W. Frank, D. M. Tanenbaum, J. M. Parpia, H. G. Craighead and P. L. McEuen, Science, 2007, 315, 490–493 CrossRef CAS.
- J. B. Oostinga, H. B. Heersche, X. Liu, A. Morpurgo and M. K. Vandersypen, Nat. Mater., 2008, 7, 151–157 CrossRef CAS.
- E. J. Duplock, M. Scheffler and P. J. D. Lindan, Phys. Rev. Lett., 2004, 92, 225502–4 CrossRef.
- Y. B. Zhang, J. P. Small, M. E. S. Amori and P. Kim, Phys. Rev. Lett., 2005, 94, 176803–4 CrossRef.
- K. S. Novoselov, A. K. Geim, S. V. Morozov, D. Jiang, M. I. Katsnelson, I. V. Grigorieva, S. V. Dubonos and A. A. Firsov, Nature, 2005, 438, 197–200 CrossRef.
- M. P. Levendorf, C. S. Ruiz-Vargas, S. Garg and J. Park, Nano Lett., 2009, 9, 4479–4483 CrossRef CAS.
- Y. X. Xu, L. Zhao, H. Bai, W. J. Hong, C. Li and G. Q. Shi, J. Am. Chem. Soc., 2009, 131, 13490–13497 CrossRef CAS.
- W. J. Hong, Y. X. Xu, G. W. Lu, C. Li and G. Q. Shi, Electrochem. Commun., 2008, 10, 1555–1558 CrossRef CAS.
- S. Alwarappan, A. Erdem, C. Liu and C. Z. Li, J. Phys. Chem. C, 2009, 113, 8853–8857 CrossRef CAS.
- Q. Liu, Z. F. Liu, X. Y. Zhong, L. Y. Yang, N. Zhang, G. L. Pan, S. G. Yin, Y. Chen and J. Wei, Adv. Funct. Mater., 2009, 19, 894–904 CrossRef CAS.
- L. H. Liu and M. Yan, Nano Lett., 2009, 9, 3375–3378 CrossRef CAS.
- S. Stankovich, R. D. Piner, X. Chen, N. Wu, S. T. Nguyen and R. S. Ruoff, J. Mater. Chem., 2006, 16, 155–158 RSC.
- S. Z. Zu and B. H. Han, J. Phys. Chem. C, 2009, 113, 13651–13657 CrossRef CAS.
- D. Li, M. B. Mu, S. Gilje, R. B. Kaner and G. G. Wallace, Nat. Nanotechnol., 2008, 3, 101–105 CrossRef CAS.
- Y. X. Xu, H. Bai, G. W. Lu, C. Li and G. Q. Shi, J. Am. Chem. Soc., 2008, 130, 5856–5857 CrossRef CAS.
- S. Stankovich, R. D. Piner, S. T. Nguyen and R. S. Ruoff, Carbon, 2006, 44, 3342–3347 CrossRef CAS.
- S. Wang, P. J. Chia, L. L. Chua, L. H. Zhao, R. Q. Png, S. Sivaramakrishnan, M. Zhou, R. G. S. Goh, R. H. Friend, A. T. S. Wee and P. K. H. Ho, Adv. Mater., 2008, 20, 3440–3446 CrossRef CAS.
- S. B. Bon, L. Valentini, R. Verdejo, J. L. G. Fierro, L. Peponi, M. A. L. Manchado and J. M. Kenny, Chem. Mater., 2009, 21, 3433–3438 CrossRef CAS.
- J. R. Lomeda, C. D. Doyle, D. V. Kosynkin, W. F. Hwang and J. M. Tour, J. Am. Chem. Soc., 2008, 130, 16201–16206 CrossRef CAS.
- P. Blake, P. D. Brimicombe, R. R. Nair, T. J. Booth, D. Jiang, F. Schedin, L. A. Ponomarenko, S. V. Morozov, H. F. Gleeson, E. W. Hill, A. K. Geim and K. S. Novoselov, Nano Lett., 2008, 8, 1704–1708 CrossRef.
- Y. Hernandez, V. Nicolosi, M. Lotya, F. M. Blighe, Z. Y. Sun, S. De, I. T. McGovern, B. Holland, M. Byrne, Y. K. Gun'ko, J. J. Boland, P. Niraj, G. Duesberg, S. Krishnamurthy, R. Goodhue, J. Hutchison, V. Scardaci, A. C. Ferrari and J. N. Coleman, Nat. Nanotechnol., 2008, 3, 563–568 CrossRef CAS.
-
(a) N. Liu, F. Luo, H. X. Wu, Y. H. Liu, C. Zhang and J. Chen, Adv. Funct. Mater., 2008, 18, 1518–1525 CrossRef CAS;
(b) J. Lu, J. Yang, J. Wang, A. Lim, S. Wang and K. P. Loh, ACS Nano, 2009, 3, 2367–2375 CrossRef CAS.
-
(a) J. Chen, M. A. Hamon, H. Hu, Y. Chen, A. M. Rao, P. C. Eklund and R. C. Haddon, Science, 1998, 282, 95–98 CrossRef CAS;
(b) E. T. Mickelson, C. B. Huffman, A. G. Rinzler, R. E. Smalley, R. H. Hauge and J. L. Margrave, Chem. Phys. Lett., 1998, 296, 188–194 CrossRef CAS;
(c) J. L. Bahr, J. Yang, D. V. Kosynkin, M. J. Bronikowski, R. E. Smalley and J. M. Tour, J. Am. Chem. Soc., 2001, 123, 6536–6542 CrossRef CAS;
(d) V. Georgakilas, K. Kordatos, M. Prato, D. M. Guldi, M. Holzinger and A. Hirsch, J. Am. Chem. Soc., 2002, 124, 760–761 CrossRef CAS.
-
(a) J. Chen, A. M. Rao, S. Lyuksyutov, M. E. Itkis, M. A. Hamon, H. Hu, R. W. Cohn, P. C. Eklund, D. T. Colbert, R. E. Smalley and R. C. Haddon, J. Phys. Chem. B, 2001, 105, 2525–2528 CrossRef CAS;
(b) R. J. Chen, Y. Zhang, D. Wang and H. Dai, J. Am. Chem. Soc., 2001, 123, 3838–3839 CrossRef CAS;
(c) A. Star, Y. Liu, K. Grant, L. Ridvan, J. F. Stoddart, D. W. Steuerman, M. R. Diehl, A. Boukai and J. R. Heath, Macromolecules, 2003, 36, 553–560 CrossRef CAS.
-
(a) M. Holzinger, O. Vostrowsky, A. Hirsch, F. Hennrich, M. Kappes, R. Weiss and F. Jellen, Angew. Chem., Int. Ed., 2001, 40, 4002–4005 CrossRef CAS;
(b) M. Holzinger, J. Abraha, P. Whelan, R. Graupner, L. Ley, F. Hennrich, M. Kappes and A. Hirsch, J. Am. Chem. Soc., 2003, 125, 8567–8580;
(c) S. J. Pastine, D. Okawa, B. Kessler, M. Rolandi, M. Llorente, A. Zettl and J. M. J. Fréchet, J. Am. Chem. Soc., 2008, 130, 4238–4239 CrossRef CAS.
- V. Sajini, J. Paul and S. Valiyaveettil, Carbon, 2009, 47, 3288–3294 CrossRef.
- J. C. Meyer, A. K. Geim, M. I. Katsnelson, K. S. Novoselov, T. J. Booth and S. Roth, Nature, 2007, 446, 60–63 CrossRef CAS.
-
J. B. Lambert, H. F. Shurvell, D. A. Lightner and R. G. Cooks, in Organic Structural Spectroscopy, Prentice Hall, Upper Saddle River, NJ, 1998, pp 175 Search PubMed.
- Y. Y. Wang, Z. H. Ni, T. Yu, Z. X. Shen, H. M. Wang, Y. H. Wu, W. Chen and A. T. S. Wee, J. Phys. Chem. C, 2008, 112, 10637–10640 CrossRef CAS.
- A. C. Ferrari, J. C. Meyer, V. Scardaci, C. Casiraghi, M. Lazzeri, F. Mauri, S. Piscanec, D. Jiang, K. S. Novoselov, S. Roth and A. K. Geim, Phys. Rev. Lett., 2006, 97, 187401–4 CrossRef CAS.
- K. L. Kelly, E. Coronado, L. L. Zhao and G. C. Schatz, J. Phys. Chem. B, 2003, 107, 668–677 CrossRef CAS.
- G. Williams, B. Seger and P. V. Kamat, ACS Nano, 2008, 2, 1487–1491 CrossRef CAS.
- N. A. Kotov, I. Dekany and J. H. Fendler, Adv. Mater., 1996, 8, 637–641 CrossRef CAS.
- Y. Si and E. T. Samulski, Nano Lett., 2008, 8, 1679–1682 CrossRef CAS.
- W. P. Heal, S. R. Wickramasinghe, P. W. Bowyer, A. A. Holder, D. F. Smith, R. J. Leatherbarrow and E. W. Tate, Chem. Commun., 2008, 480–482 RSC.
|
This journal is © The Royal Society of Chemistry 2011 |
Click here to see how this site uses Cookies. View our privacy policy here.