DOI:
10.1039/C1RA00014D
(Paper)
RSC Adv., 2011,
1, 415-423
Synthesis, characterization and application of starburst 9-alkyl-1,3,6,8-tetraaryl-carbazole derivatives for blue-violet to UV OLEDs†
Received
4th April 2011
, Accepted 6th June 2011
First published on 9th August 2011
Abstract
A series of starburst carbazole derivatives, which comprise various aryl groups appended to the 1,3,6,8-positions of a 9-alkyl-carbazole core, are synthesized and characterized. These compounds exhibit good thermal stabilities with glass transition temperatures up to 199 °C and thermal decomposition temperatures ranging from 321 to 485 °C. The starburst configuration of the as-prepared compounds results in fluorescence emission in the UV to blue-violet region (λmax = 389–409 nm) with photoluminescence quantum efficiency (ΦPL) reaching 90% and narrow full width half maximum (FWHM) of 43–54 nm in solution. Light-emitting devices are successfully fabricated using these materials as emitters, and emit UV to blue-violet light. With a device structure of indium tin oxide (ITO)/molybdenum trioxide (MoO3)/1,3,6,8-tetraaryl carbazole derivatives/1,3,5-tris(2-N-phenylbenzimidazolyl)benzene (TPBI)/8-hydroxyquinoline aluminum (Alq3)/LiF/Al, maximum external quantum efficiency (EQEmax) of 2.05% and 3.4% have been obtained at UV wavelength of 394 nm with FWHM of 42 nm and blue-violet wavelength of 415 nm with FWHM of 46 nm, respectively.
1. Introduction
Organic light-emitting devices (OLEDs) have been attracting a great deal of scientific and commercial interest owing to their promising application in flat-panel displays1,2 and solid-state illumination sources3,4 since the first efficient OLED was reported.5 Studies on the three primary colors have made remarkable progress for OLEDs in past decade. Recently, there has been even greater interest in developing OLEDs that emit light of shorter wavelengths, i.e., blue-violet to UV, since such devices can be utilized to generate full-color OLED displays by irradiation of luminescent dyes; applied to a white light source by using red, green and blue color conversion materials;6 functioned as host material for electrophosphorescence;7,8 used in disinfection systems;9 serving as a potential light source for high-density information storage devices10–12 and fluorescent sensors.13
As is well known, it is difficult to obtain promising materials for fabricating OLEDs with emission from blue-violet to UV because the materials possess a large band gap, which makes it hard to inject charges into the emitters. Furthermore, the effective conjugation length in these materials is short. This directly reduces the conductivity and impacts the performance of OLEDs. However, there have been some attempts to develop blue-violet to UV organic electroluminescence (EL) devices, such as 2-(4-biphenylyl)-5-(p-tert-butylphenyl)-1,3,4-oxadiazole (PBD) [ELλmax = 394 nm; external quantum efficiency (EQE) = 0.1%],144,4′-bis(carbazol-9-yl)biphenyl (CBP) (ELλmax = 390 nm; EQE = 1%),15N,N′-bis(3-methylphenyl)- N,N′-diphenyl-(1,1′-biphenyl)-4,4′-diamine (TPD) (ELλmax = 404 nm; EQE = 1.4%)16 and polysilane (ELλmax = 405 nm; EQE = 0.2%).17 Up to now, only bi(9,9-diaryfluorene)s (ELλmax = 374, 392 nm; EQE = 3.6%)18 and 3,5-dophenyl-4-napth-1-yl-1,2,4-triazol (TAZ) (ELλmax = 380 nm; EQE = 3.1%)6 have been reported to emit UV light with an EQE higher than 3%. However, further improvement in both the EQE and color purity of OLEDs is still necessary. Therefore, developing new blue-violet to UV emitting materials with excellent performance is desirable.
Recently, both carbazole-based small molecules and polymers have been extensively investigated as efficient host materials, blue emitters and hole-transporters for applications in OLEDs.19–25 Thereinto, the derivatives with a carbazole unit as central core were prepared by functionalizing at its 3,6-positions, 2,7-positions or 9-position.26–30 The as-prepared compounds with thermally and morphologically stable properties can expand the application of carbazole in OLEDs. Simultaneously, the starburst molecules are composed of multi-conjugated arms, which stretch in different orientations. Due to their thermal stability, good film-forming properties and high luminescence,31,32 the starburst molecules have attracted much more attention to the application in OLEDs, photovoltaics and field-effect transistors.33–36 Based on the above-mentioned, designing carbazole derivatives with a star-configuration leads to an angle between the carbazole core and peripheral substituents, which can effectively confine the conjugated structure and emit shorter wavelength light. Therefore, developing starburst derivatives with a carbazole unit at the core will provide promising materials for excellent UV OLEDs. In this study, we report a series of carbazole derivatives by using carbazole as the central core and functionalizing at its 1,3,6,8-positions with various aryl moieties. Herein, the optical, electrochemical, thermal and EL properties of the as-prepared carbazole derivatives were also investigated.
2. Results and discussion
2.1. Material synthesis
The synthetic routes to the starburst 1,3,6,8-tetraaryl carbazole derivatives are outlined in Scheme 1. To a solution of 9-alkyl-9H-carbazole in glacial acetic acid, a solution of bromine in glacial acetic acid was added. Then the 1,3,6,8-tetrabromo-9-alkyl-9H-carbazoles (A, B and C) were obtained. All of the desired compounds were readily obtained by a one-pot Suzuki cross-coupling reaction using A, B and C with commercially available boronic acids and borates which were used without further purification. In order to have a comprehensive study of substituent effects on the properties, compounds 1–12 were synthesized with 9-alkyl chains of different lengths and different aryl substituents as branches. All the newly obtained carbazole derivatives were fully characterized by 1H NMR, 13C NMR, MS and elemental analysis.
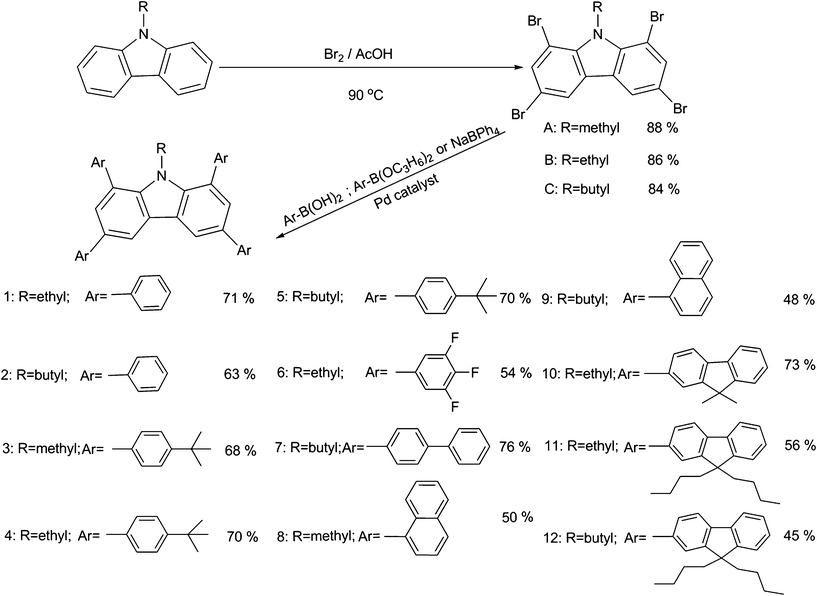 |
| Scheme 1 The synthetic procedure of compounds 1–12. | |
2.2. Photophysical properties
The photophysical properties of these compounds are summarized in Table 1. The normalized absorption spectra and photoluminescence (PL) spectra of compounds 1, 4, 6, 7, 9, 10 and 11 as representative in dilute chloroform solution are shown in Fig. 1a and 1b, respectively. It can be seen that the absorption spectra of compounds 1, 4 and 6 are composed of two major absorption bands as shown in Fig. 1a. The latter absorption can be assigned to the transitions in the carbazole core.37 On the other hand, the absorption maxima of compounds 7, 9, 10 and 11 fall within 294–322 nm, They display absorptions resulting from the combination of carbazole and diphenyl, naphthalene, or fluorene chromophores.38 Meanwhile, Fig. 1b illustrates that all the compounds exhibit UV to blue-violet emission with emission maxima from 389 nm to 409 nm. As shown in Table 1, the photoluminescence quantum yields (ΦPL) are in the range 25–90%. Moreover, all the compounds exhibit good color purity with narrow FWHM in the range 43–54 nm. Therefore, the as-prepared carbazole derivatives can be used as excellent candidates for the blue-violet to UV OLEDs.
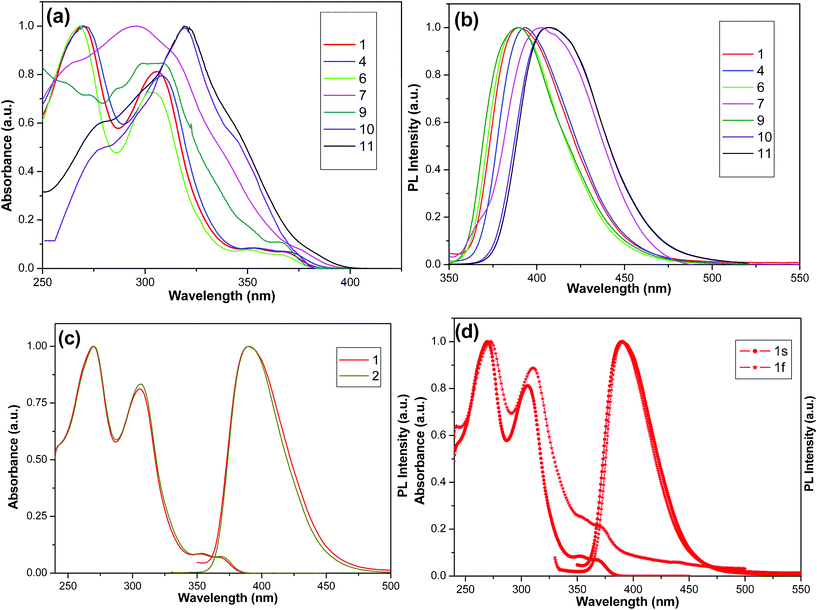 |
| Fig. 1 (a) Normalized absorption spectra of compounds 1, 4, 6, 7, 9, 10 and 11 in chloroform solution. (b) Normalized PL spectra of compounds 1, 4, 6, 7, 9, 10 and 11 in chloroform solution. (c) Normalized absorption spectra and PL spectra of compounds 1 and 2 in chloroform solution. (d) Normalized absorption spectra and PL spectra of compound 1 in chloroform solution (1s) and as a thin film (1f). | |
Table 1 Physical data for compounds 1–12
Compound |
λ
abs
a[nm] |
λ
em
(ΦPL)b[nm] |
FWHMc[nm] |
T
g
d[°C] |
T
d
e[°C] |
E
g
f[eV] |
HOMO/LUMOg[eV] |
Measured in CHCl3.
Measured in CHCl3, using quinine (0.05 mol L−1sulfate) (ΦPL = 0.55) as a standard.
FWHM: full width half maximum.
Obtained by DSC under N2.
Obtained by TGA under N2.
Band gaps were calculated from the onset at the long wavelength end of the absorption spectrum.
HOMO (highest occupied molecular orbital) levels calculated with reference to ferrocene (4.8 eV). LUMO (lowest occupied molecular orbital) levels were estimated by subtracting the Eg from HOMO levels.
|
1
|
367,354,306,270 |
390(0.56) |
47 |
93 |
341 |
3.25 |
5.06/1.81 |
2
|
372,358,307,270 |
390(0.47) |
45 |
78 |
335 |
3.22 |
5.05/1.83 |
3
|
372,361,310,280 |
393(0.48) |
43 |
163 |
387 |
3.14 |
5.08/1.94 |
4
|
355,309,271 |
393(0.49) |
46 |
165 |
390 |
3.15 |
5.02/1.87 |
5
|
370,356,307,271 |
392(0.25) |
46 |
153 |
383 |
3.20 |
5.01/1.81 |
6
|
302,268 |
389(0.51) |
46 |
107 |
321 |
3.22 |
5.11/1.89 |
7
|
367,309 |
396(0.53) |
44 |
165 |
436 |
3.23 |
5.01/1.78 |
8
|
375,299 |
395(0.51) |
51 |
125 |
396 |
3.26 |
5.06/1.80 |
9
|
294 |
409(0.66) |
51 |
112 |
363 |
3.02 |
5.04/1.90 |
10
|
319 |
407(0.90) |
54 |
199 |
485 |
3.14 |
5.08/1.99 |
11
|
320 |
407(0.87) |
53 |
134 |
428 |
3.09 |
5.03/1.92 |
12
|
322 |
405(0.77) |
53 |
120 |
410 |
3.11 |
5.03/1.92 |
By combining the optical spectra with the composition of these carbazole derivatives, several conclusions can be obtained. Firstly, the absorption spectra and PL spectra are affected by the properties of peripheral substituents. As shown in Fig. 1a and 1b, with the same N-ethyl of compounds 1, 4 and 6, compared to the absorption and emission spectra of compound 1, only small red-shifts resulting from the electron-donating nature of the tertbutyl group was observed for compound 4, and only a small blue-shift ascribed to the electron-withdrawing nature of fluorine was present in compound 6. Secondly, the length of the alkyl chain of the N-alkyl substituent has little effect on the photophysical properties. Fig. 1c shows the normalized absorption spectra and PL spectra of compounds 1 and 2 in chloroform solution. It demonstrates that the normalized absorption spectra and fluorescence spectra of these compounds basically remain the same when the compounds are composed of the same peripheral phenyl substituents and the N–H substituents were replaced by an N-ethyl (1) and N-butyl (2) group. Last but not the least, the ΦPL depends on different aryl substituents. Compounds 10, 11 and 12 functionalized with a peripheral fluorene chromophore possess high ΦPL, up to 90%.
Fig. 1d shows the normalized absorption and PL spectra of compound 1 in chloroform solution and as a thin film. It can be seen that the normalized absorption spectra of compound 1 in the solid state is similar to that in solution. The thin film absorption spectra are broader and red-shifted by 3 nm compared to that in dilute solution. This slight change can be ascribed to the increased electron delocalization in the solid state.
The normalized PL spectra of compound 1 in the solid state is almost the same as that in solution. The red-shift of the PL emission maxima by only 1 nm is of the same origin as the changes in the absorption spectra, increased delocalization in the solid state. But this effect is not obvious.
To better understand the relationship between structure and properties, a single crystal of compound 6, which is suitable for the determination of X-ray crystal structure, was grown by slow evaporation of tetrahydrofuran (THF)/hexane solution. The crystal structure is shown in Fig. 2. It shows that it is in the form of a primitive monoclinic crystal and its space group is P21/c. It is clearly seen that it possesses an essentially planar core, carbazole ring (Fig. 2a). Thereinto, the solvent molecule (THF) as ligand is entered into the crystal structure. The 3,6-phenyl groups are only slightly distorted at angles of 36 and 27° relative to the carbazole moiety and the 1,8-phenyl groups are distorted at large angles of 55 and 50° relative to the planar carbazole ring, which caused a distinct bulge. It is essential in preventing molecules from close packing and hence undergoing severe fluorescence quenching. Fig. 2b shows the crystal-packing diagram of compound 6. The closest intermolecular C–C distance between the carbazole planes of two adjacent molecules is about 6.46 Å (c/2). The result can be attributed to the effect of 1,3,6,8-aryl groups in the crystal packing. There is no efficient plan-to-plan π–π interaction between the carbazole planes of two adjacent molecules in the crystal. Therefore, the driving force for the molecular stacking is mainly an intermolecular van der Waals force. One may surmise that the excition transfer is limited or eliminated and the concentration-quenching effect may be weakened in such structures.39,40
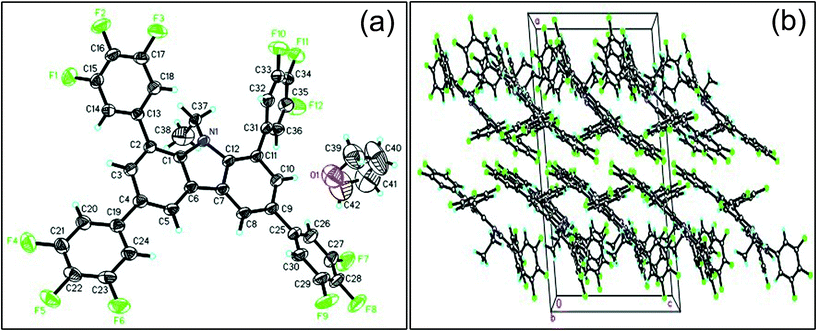 |
| Fig. 2 (a) The crystal structure of compound 6. (b) The crystal-packing diagram of compound 6. | |
2.4. Thermochemical properties
The thermal properties of these compounds were investigated by differential scanning calorimetry (DSC) and thermogravimetric analysis (TGA). The pertinent data are presented in Table 1. The TGA experiments suggest that all these starburst compounds exhibit excellent thermal stabilities, with decomposition temperatures (Td) in the range 321–485 °C (weight losses of 5%). The DSC results reveal that all the compounds exhibit a glass transition in the second heating cycle and the glass transition temperature (Tg) reaches as high as 199 °C . Additionally, the melting points of the compounds were determined by using a heating rate of 10 °C min−1 in the first heating cycle. For the 1,3,6,8-tetraaryl carbazole derivatives in this study, with the same peripheral aryl substituent, replacement of the N-H substituent by an N-ethyl (1, 4 and 11) and N-butyl (2, 5 and 12), the Tg and Td (1 > 2, 4 > 5 and 11 > 12) decrease as the length of the alkyl chain increases. Furthermore, with the same N-butyl (2, 5, 7 and 9), the Tg and Td increase with the size of the 1,3,6,8-tetraaryl substituents attached to the carbazole core as follows: 4-biphenyl (7) > 4-tert-butyl-phenyl (5) > 1-naphthyl (9) > phenyl (2). Accordingly, the trends suggest that a shorter alkyl chain and higher molecular weight can improve the thermal stability.37,39,41,42
2.5. Electrochemical properties
Cyclic voltammetry (CV) experiments were performed to investigate the electrochemical properties of these compounds. The CV curve of compound 10, as a representative, suggests good reversible oxidation waves with two similar reversible oxidation processes as show in Fig. 3. The first and second oxidation occur around 0.7 V and 1.0 V, which can be attributed to the simultaneous multielectron oxidation process of the four peripheral aryl substituents resulting in radical cations and the removal of electrons from the carbazole core generating diradical cations.43 The highest occupied molecular orbital (HOMO) (−5.11 to −5.01 eV) levels of these compounds were calculated by using oxidation onset potentials (Table 1). On the other hand, the lowest unoccupied molecular orbital (LUMO) (−1.99 to −1.78 eV) levels were deduced from the difference between the HOMO levels and the bandgaps obtained from the absorption spectra (Table 1). In addition, The HOMO levels increase systematically from −5.11 to −5.02 eV, as the 1,3,6,8-tetraaryl substituents are changed from electron-withdrawing (6) to electron-donating substituents (4). These calculated HOMO levels for the compounds allow a lower barrier for hole injection from the indium tin oxide (ITO) anode. Therefore, the starburst carbazole derivatives can be used as promising hole-transport materials for OLEDs.
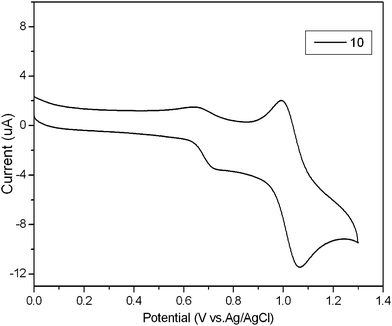 |
| Fig. 3
Oxidation part of the CV curve of compound 10, measured in MeCN and CH2Cl2 (1 : 1, v/v) containing Bu4NPF6 (0.1 M) as supporting electrolyte. | |
2.6. Electroluminescent properties
According to the HOMO and LUMO data (Table 1), all the compounds in this study appear to be good candidates for hole-transporting and emitting materials, which is consistent with the excellent capabilities of reported carbazole derivatives.44 From the PL results in Fig. 1b, the 1-, 4- and 10-based devices are expected to emit UV to blue-violet light in OLEDs. But to the poor electron-injection and electron-transport capabilities of carbazole derivatives, a hole-blocking layer, TPBI, was inserted between the emitting layer and electron-transporting layer (Alq3). For an impartial comparison, four non-doped OLEDs have the same simple configuration: ITO/MoO3 (5 nm)/emitter (30 nm)/TPBI (10 nm)/Alq3 (40 nm)/LiF (1 nm)/Al (100 nm), where compounds 1, 4, 10 and TPD are used as the emitting layers (Fig. 4).
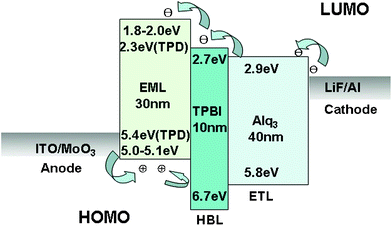 |
| Fig. 4 The device structure and materials used in this study. | |
The performance characteristics of these four devices are summarized in Table 2 and Fig. 5. Herein, the electroluminescence data of device TPD is comparable to the value reported in the literature for OLEDs with TPD as an emitter.16,45 As shown in Fig. 5a, all EL spectra of these 1-, 4- and 10-based devices display a UV to blue-violet light emission. Their emission maxima are 394, 395 and 415 nm, respectively, which correspond well with the PL spectra in solution (Fig. 1b). Thus, it clearly demonstrates that the EL peaks from these devices originated from compounds 1, 4 and 10. Furthermore, they show quite narrow FWHM of about 41–46 nm which are narrower than the data measured in dilute chloroform solution. Besides that, the FWHM of these OLEDs are also obviously narrower than that in other reports.14–16,46–49 These high color purity emissions confirm that the non-coplanar molecular structures of the three compounds can effectively hinder intermolecular aggregation.
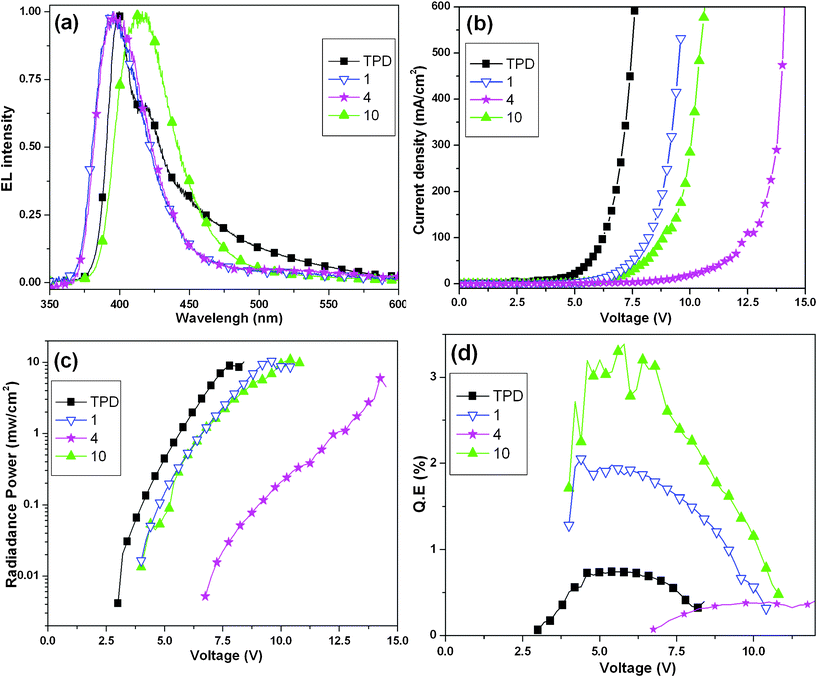 |
| Fig. 5 (a) EL spectra. (b) Current density–voltage characteristics. (c) Radiance–voltage characteristics. (d) EL quantum efficiency versus voltage characteristics. | |
Table 2 Electroluminescence data of the devices for compounds 1, 4, 10 and TPD
Compound |
V
on
a [V] |
λ
em [nm] |
FWHM [nm] |
η
ext, max
b [%] |
V
on: turn-on voltage.
η
ext: external quantum efficiency.
|
TPD
|
3.2 |
399 |
50 |
0.73 |
1
|
4 |
394 |
42 |
2.05 |
4
|
7 |
395 |
41 |
0.4 |
10
|
4 |
415 |
46 |
3.4 |
According to Fig. 5b and 5c, it can be seen that the 1- and 10-based devices have a low turn-on voltage (4 V) and the value of 4-based device is 7 V. In assessing the performance of these devices, the 1-based device emits a radiance of 10.4 mW cm−2 at 9.6 V which show a high performance with hitherto reported UV OLEDs with peak below 400 nm.14,15 Additionally, the 10-based device with the best of blue-violet light emission emits a radiance of 10.9 mW cm−2 at 10.4 V. Compared with the 1- and 10-based devices, the performances of 4-based devices is not well, which is 0.2 mW cm−2 at 10.0 V. Fig. 5d illustrates the maximum external quantum efficiencies (EQEmax) of these devices versus applied voltage. The EQE starts to increase after the device is lighted on and reaches a maximum near 6 V. Compared to earlier reports,50,51 compound 10 combines characteristics of both carbazole and fluorene. Furthermore, compound 10 was simultaneously used as hole-transport and emitting layers, TPBI was used as a hole-blocking layer to confine the charge-recombination region in the emitting layer in the device. Therefore, the EQEmax of 10-based device was up to 3.4% at blue-violet wavelength of 415 nm. Anyhow, it is still worthy to note that the values of EQEmax are among the best ever reported in the literature for UV to blue-violet nondoped OLEDs45–47,52,53 and even doped OLEDs.14,48
3. Conclusions
In conclusion, a series of 1,3,6,8-tetraaryl carbazole derivatives with a starburst configuration was synthesized by a Suzuki cross-coupling reaction. These new compounds possess excellent thermal stabilities (Td = 321–485 °C) and display UV to blue-violet fluorescence (λmax = 389–409 nm) in solution with narrow FWHM (43–54 nm). Furthermore, the compounds were used as emitting materials to fabricate the nondoped OLED devices which displayed UV and blue-violet light emission with maximum EQE of 2.04% and 3.4%, respectively. The results show that the prepared carbazole derivatives could be useful for the efficient UV and blue-violet organic light emitting diodes. Based on this work, investigations of other more short-wavelength materials are currently underway in our laboratory.
4. Experimental
4.1. General procedures
All chemical reagents were used as received from commercial sources without further purification unless otherwise stated. Solvents for chemical synthesis and measurement were purified according to the standard procedures. All reactions were monitored by thin-layer chromatography (TLC) and compounds were visualized with UV light irradiation at 254 and 365 nm. Column chromatography was carried out using silica gel (300–400 mesh). 1H and 13C NMR spectra were recorded using Brüker AVANCE 300M, 400M and 500M spectrometer. Time-of-flight (TOF), matrix-assisted laser desorption ionization time-of-flight (MALDI-TOF), fast atom bombardment (FAB) mass spectra were measured with a Waters GC-EI/CI time-of-flight mass spectrometer, a Brüker BIFLEXIII and a Thermo DSQ EI-mass spectrometer. Elemental analyses were taken with Elementar vario EL elemental analyzer. X-Ray single-crystal diffraction analysis was performed on SMART-APEX equipment at the Shanghai Institute of Organic Chemistry (China Academy of Science). UV-vis spectra were obtained using a Shimadzu UV-2501PC while PL spectra were taken using a Perkin Elmer LS-45 fluorescence spectrophotometer. Emission quantum yields were measured in CHCl3 solutions at 293 K against quinine (0.05 mol L−1sulfate) as reference.54DSC analyses were performed using a Netzsch DSC-204 differential scanning calorimeter operated at heating and cooling rates of 10 °C and 70 °C min−1, respectively. Melting points were determined from the second heating scan. TGA measurements were performed on a Netzsch TG-209 thermogravimeter under a dry nitrogen gas flow at a heating rate of 10 °C min−1. Cyclic voltammetry measurements were carried out using a CHI660A electrochemical workstation at room temperature with conventional three-electrode configuration, which consisted of a platinum plate working electrode, an Ag/AgCl reference electrode and a platinum plate counter electrode. CV was carried out in MeCN and CH2Cl2 (1
:
1, v/v) containing Bu4NPF6 (0.1 M) as supporting electrolyte. The electrolyte was deaerated by N2 (99.99%) for 5 min in advance. Ferrocene was added as a standard and the ferrocene/ferrocenium ion (Fc/Fc+) redox couple shows the half-wave potential (E1/2) at 0.46 V in the same measurement condition, which means that the potential of the Ag/AgCl is −4.34 eV relative to the oxidation potential of ferrocene at −4.8 eV below vacuum.55
4.2. Synthesis
1,3,6,8-Tetrabromo-9-methyl-9H-carbazole (A).
9-Methyl-9H-carbazole (2.72 g, 15 mmol) was dissolved in glacial acetic acid (60 mL) in a three-necked 100 mL round-bottom flask. With stirring at room temperature, a solution of bromine (68 mmol) in glacial acetic acid (40 mL) was then added slowly. The mixture was stirred and heated at 90 °C for 10 h. After cooling to room temperature and standing overnight, compound A was collected by filtration and recrystallized from toluene/glacial acetic acid. White solid. Yield: 88%. mp 192.7 °C. 1H NMR (CDCl3, 500 MHz), δ (ppm): 4.46 (s, 3H), 7.77 (d, J = 2.0 Hz, 2H), 8.02 (d, J = 2.0 Hz, 2H). 13C NMR (CDCl3, 500 MHz), δ (ppm): 35.63, 104.62, 113.11, 122.09, 126.13, 134.64, 138.80. TOF MS: m/z 497 (M+).
1,3,6,8-Tetrabromo-9-ethyl-9H-carbazole (B).
B was prepared by a similar method to A. White solid. Yield: 86%. mp 174.0 °C. 1H NMR (CDCl3, 500 MHz), δ (ppm): 1.42 (t, J = 7.0 Hz, 3H), 5.24 (p, J = 7.0 Hz, 2H), 7.80 (s, 2H), 8.06 (s, 2H). 13C NMR (CDCl3, 500 MHz), δ (ppm): 17.19, 39.70, 104.02, 112.87, 122.05, 126.29, 134.96, 136.81. TOF MS: m/z 511 (M+).
1,3,6,8-Tetrabromo-9-butyl-9H-carbazole (C).
C was prepared by a similar method to A. White solid. Yield: 84%. mp 170.5 °C. 1H NMR (CDCl3, 500 MHz), δ (ppm): 0.95 (t, J = 7.0 Hz, 3H), 1.31–1.39 (m, 2H), 1.73–1.79 (m, 2H), 5.14 (t, J = 8.0 Hz, 2H), 7.79 (d, J = 1.5 Hz, 2H), 8.05 (d, J = 1.5 Hz, 2H). 13C NMR (CDCl3, 300 MHz), δ (ppm): 13.84, 19.41, 33.82, 44.50, 104.23, 112.77, 122.05, 126.23, 134.92, 137.04. TOF MS: m/z 539 (M+).
9-Ethyl-1,3,6,8-tetraphenyl-9H-carbazole (1).
A mixture of B (1.02 g, 2 mmol), sodium tetraphenylboron (856 mg, 2.5 mmol), TEBA (45.55 mg, 0.2 mmol) and palladium chloride (7 mg, 0.04 mmol) in acetone was flushed with nitrogen. Then the Na2CO3 (2 M) aqueous solution (12 mL) were added. The resultant mixture was stirred at 80 °C for 12 h. After cooling to room temperature, the solvent was removed under vacuum and the residue was extracted with dichloromethane, washed with water and dried over MgSO4. The solvent was evaporated and product was isolated by column chromatography using silica gel with a mixture of petroleum ether and dichloromethane. White solid. Yield: 63%. mp 210.0 °C. 1H NMR (CDCl3, 500 MHz), δ (ppm): 0.38 (t, J = 6.8 Hz, 3H), 3.54 (p, J = 6.5 Hz, 2H), 7.36–7.43 (m, 4H), 7.47–7.52 (m, 8H), 7.58 (s, 2H), 7.62 (d, J = 6.0 Hz, 4H), 7.80 (d, J = 6.0 Hz, 4H), 8.43 (d, J = 1.9 Hz, 2H). 13C NMR (CDCl3, 500 MHz), δ (ppm): 13.57, 40.29, 117.54, 126.65 (double), 127.25, 127.36, 127.52, 128.23, 128.52, 128.76, 129.25, 133.24, 139.42, 140.66, 141.54.MALDI-TOF: m/z 499 (M+). Anal. Calcd for C38H29N: C 91.35, H 5.85, N 2.80; found: C 91.59, H 5.99, N 2.80.
9-Butyl-1,3,6,8-tetraphenyl-9H-carbazole (2).
2 was prepared by a similar method to 1. White solid. Yield: 71%. mp 173.1 °C. 1H NMR (CDCl3, 500 MHz), δ (ppm): 0.27–0.35 (m, 5H), 0.83–0.89 (m, 2H), 3.43 (t, J = 7.8 Hz, 2H), 7.33–7.40 (m, 4H), 7.44–7.49 (m, 8H), 7.55–7.58 (m, 6H), 7.77 (d, J = 7.5 Hz, 4H). 8.40 (d, J = 1.5 Hz, 2H). 13C NMR (CDCl3, 500 MHz), δ (ppm): 13.21, 19.14, 30.78, 45.47, 117.53, 126.21, 126.62, 127.25, 127.34 (double), 128.17, 128.53, 128.76, 129.45, 132.98, 139.50, 140.70, 141.56. FAB MS: m/z 527 (M+). Anal. Calcd for C40H33N: C 91.04, H 6.30, N 2.65; found: C 90.83, H 6.47, N 2.60.
9-Methyl-1,3,6,8-tetrakis-(4-tert-butyl-phenyl)-9H-carbazole (3).
3 was prepared by a similar method to 1. White solid. Yield: 68%. mp 296.8 °C. 1H NMR (CDCl3, 500 MHz), δ (ppm): 1.40 (s, 36H), 3.00 (s, 3H), 7.50 (p, J = 8.0 Hz, 12H), 7.61 (s, 2H), 7.71 (d, J = 7.5 Hz, 4H), 8.36 (S, 2H). 13C NMR (CDCl3, 500 MHz), δ (ppm): 31.45, 31.48, 34.52, 34.64, 37.56, 117.14, 124.97, 125.52, 125.68, 126.76, 126.91, 128.61, 129.29, 132.83, 137.60, 138.83, 140.68, 149.55, 150.14. FAB MS: m/z 710 (M+). Anal. Calcd for C53H59N: C 89.65, H 8.38, N 1.97; found: C 89.50, H 8.20, N 1.97.
9-Ethyl-1,3,6,8-tetrakis-(4-tert-butyl-phenyl)-9H-carbazole (4).
4 was prepared by a similar method to 1. White solid. Yield: 70%. mp 326.2 °C. 1H NMR (CDCl3, 400 MHz), δ (ppm): 0.39 (t, J = 7.0 Hz, 3H), 1.39 (d, J = 0.8 Hz, 36H), 3.63 (p, J = 7.0 Hz, 2H), 7.46–7.53 (m, 12H), 7.54 (d, J = 1.6 Hz, 2H), 7.70 (d, J = 8.4 Hz, 4H), 8.35 (d, J = 1.6 Hz, 2H). 13C NMR (CDCl3, 300 MHz), δ (ppm): 13.62, 31.43, 31.44, 34.49, 34.62, 40.27, 117.09, 125.01, 125.67, 126.58, 126.86, 127.31, 128.68, 128.88, 132.88, 137.70, 138.72, 139.26, 149.49, 150.18. MALDI-TOF: m/z 723.5 (M+). Anal. Calcd for C54H61N: C 89.57, H 8.49, N 1.93; found: C 89.32, H 8.39, N 1.94.
9-Butyl-1,3,6,8-tetrakis-(4-tert-butyl-phenyl)-9H-carbazole (5).
5 was prepared by a similar method to 1. White solid. Yield: 70%. mp 281.5 °C. 1H NMR (CDCl3, 300 MHz), δ (ppm): 0.18–0.26 (m, 5H), 0.84–0.94 (m, 2H), 1.38 (d, J = 4.2 Hz, 36H), 3.48 (t, J = 8.0 Hz, 2H), 7.45–7.50 (m, 12H), 7.52 (d, J = 1.8 Hz, 2H), 7.70 (d, J = 8.4 Hz, 4H), 8.37 (d, J = 1.8 Hz, 2H). 13C NMR (CDCl3, 500 MHz), δ (ppm): 12.95, 17.57, 19.08, 31.41, 31.44, 34.49, 34.61, 45.19, 117.08, 124.77, 125.59, 125.65, 126.83, 128.58, 129.34, 132.23, 137.81, 138.73, 149.45, 150.24. FAB MS: m/z 751 (M+). Anal. Calcd for C56H65N: C 89.43, H 8.71, N 1.86; found: C 89.19, H 8.73, N 1.80.
9-Ethyl-1,3,6,8-tetrakis-(3,4,5-trifluoro-phenyl)-9H-carbazole (6).
6 was prepared by a similar method to 1. White solid. Yield: 54%. mp 288.0 °C. 1H NMR (CDCl3, 300 MHz), δ (ppm): 0.50 (t, J = 7.1 Hz, 3H), 3.58 (p, J = 6.9 Hz, 2H), 7.22 (t, J = 7.1 Hz, 4H), 7.31–7.36 (m, 4H), 7.42 (d, J = 1.8 Hz, 2H), 8.31 (d, J = 1.8 Hz, 2H). 13C NMR (CDCl3, 500 MHz), δ (ppm): 13.48, 40.91, 110.92, 111.21, 113.28, 113.46, 113.56, 115.60, 118.56, 124.88, 126.81, 128.17, 131.15, 136.31, 139.55, 140.73. MALDI-TOF: m/z 715.3 (M+). Anal. Calcd for C38H17F12N: C 63.79, H 2.39, F 31.86, N 1.96; found: C 64.14, H 2.92, N 1.91.
9-Butyl-1,3,6,8-tetrakis-biphenyl-4-yl-9H-carbazole (7).
7 was prepared by a similar method to 1. White solid. Yield: 76%. mp 189.2 °C. 1H NMR (CDCl3, 300 MHz), δ (ppm): 0.35 (d, J = 2.7 Hz, 5H), 0.86 (m, 2H), 3.58 (t, J = 7.5 Hz, 2H), 7.34–7.40 (m, 4H), 7.45–7.50 (m, 8H), 7.67–7.75 (m, 22H), 7.89 (dd, J1 = 7.8 Hz, J2 = 2.7 Hz, 4H), 8.49 (t, J = 7.5 Hz, 2H). 13C NMR (CDCl3, 300 MHz), δ (ppm): 13.26, 19.21, 30.79, 45.68, 117.51, 126.28, 126.88, 127.01, 127.06, 127.21, 127.37, 127.42, 127.52, 128.35, 128.79, 128.83, 129.80, 132.53, 139.47, 139.72, 140.25, 140.35, 140.65, 140.82. FAB MS: m/z 831 (M+). Anal. Calcd for C64H49N: C 92.38, H 5.94, N 1.68; found: C 92.01, H 6.21, N 1.77.
9-Methyl-1,3,6,8-tetra-naphthalen-1-yl-9H-carbazole (8).
8 was prepared by a similar method to 1. White solid. Yield: 50%. mp 148.2 °C. 1H NMR (CDCl3, 300 MHz), δ (ppm): 2.39 (dd, J1 = 9.9 Hz, J2 = 4.8 Hz, 3H), 7.40–7.48 (m, 10H), 7.54–7.56 (m, 4H), 7.59–7.69 (m, 6H), 7.78–7.90 (m, 8H), 8.17 (t, J = 7.5 Hz, 2H), 8.41 (d, J = 1.5 Hz, 2H), 13C NMR (CDCl3, 500 MHz), δ (ppm): 33.98, 34.10, 120.98, 121.01, 123.79, 123.98, 124.02, 124.10, 125.08, 125.41, 125.64, 125.82, 125.93, 126.00, 126.30 (double), 126.42, 126.51, 127.32, 127.47, 127.74, 127.86, 128.10, 128.17, 128.28, 131.91, 131.94, 131.98, 132.03, 132.18 (double), 132.99, 133.13, 133.29, 134.00, 137.81, 137.97, 140.18, 140.21, 140.52, 140.54. FAB MS: m/z 685 (M+). Anal. Calcd for C53H35N: C 92.81, H 5.14, N 2.04; found: C 92.34, H 5.63, N 2.02.
9-Butyl-1,3,6,8-tetra-naphthalen-1-yl-9H-carbazole (9).
9 was prepared by a similar method to 1. White solid. Yield: 48%. mp 130.8 °C. 1H NMR (CDCl3, 300 MHz), δ (ppm): −0.18–(−0.12) (m, 2H), 0.06 (s, 2H), 0.87–0.91 (m, 3H), 2.75–2.78 (m, 2H), 7.35–7.51 (m, 10H), 7.53–7.60 (m, 4H), 7.61–7.75 (m, 6H), 7.82–7.94 (m, 8H), 8.21 (t, J = 7.5 Hz, 2H), 8.46 (s, 2H),. 13C NMR (CDCl3, 300 MHz), δ (ppm): 12.64, 13.00, 18.69, 18.81, 32.06, 32.28, 44.82, 45.34, 120.90, 120.94, 123.83, 124.15, 124.69, 125.00, 125.09, 125.40, 125.62, 125.86, 125.91, 125.97, 126.17, 126.22, 126.27, 126.48, 127.13, 127.27, 127.38, 127.45, 127.88, 127.93, 128.10, 128.14, 128.25, 131.73, 131.94, 132.03, 132.08, 132.83, 132.97, 133.27, 133.90, 137.69, 137.90, 139.03, 140.42, 140.45. FAB MS: m/z 727 (M+). Anal. Calcd for C56H41N: C 92.40, H 5.68, N 1.92; found: C 92.14, H 5.76, N 1.93.
9-Ethyl-1,3,6,8-tetrakis-(9,9-dimethyl-9H-fluoren-2-yl)-9H-carbazole (10).
10 was prepared by a similar method to 1. White solid. Yield: 73%. mp 350.9 °C. 1H NMR (CDCl3, 400 MHz), δ (ppm): 0.41 (t, J = 7.2 Hz, 3H), 1.53 (d, J = 3.2 Hz, 12H), 1.59 (d, J = 8.8 Hz, 12H), 3.53 (p, J = 6.8 Hz, 2H), 7.30–7.40 (m, 8H), 7.44–7.49 (m, 4H), 7.62 (d, J = 6.8 Hz, 2H), 7.70–7.76 (m, 6H), 7.77–7.86 (m, 8H), 7.86 (d, J = 2.8 Hz, 2H), 8.52 (s, 2H), 13C NMR (CDCl3, 500 MHz), δ (ppm): 13.75, 27.16, 27.36, 40.26, 46.94, 47.05, 117.65, 119.74, 119.98, 120.04, 120.32, 121.53, 122.59, 123.70, 126.41, 126.84, 127.00, 127.06 (double), 127.39, 128.04, 128.08, 128.39, 133.78, 137.92, 138.56, 138.80, 139.09, 139.51, 140.89, 153.70, 153.72, 153.94, 154.41. MALDI-TOF: m/z 963.6 (M+). Anal. Calcd for C74H61N: C 92.17, H 6.38, N 1.45; found: C 92.62, H 6.54, N 1.28.
9-Ethyl-1,3,6,8-tetrakis-(9,9-dibutyl-9H-fluoren-2-yl)-9H-carbazole (11).
11 was prepared by a similar method to 1. White solid. Yield: 56%. mp 155.0 °C. 1H NMR (CDCl3, 500 MHz), δ (ppm): 0.36 (t, J = 7.0 Hz, 8H), 0.52 (t, J = 7.5 Hz, 3H), 0.70 (t, J = 7.5 Hz, 24H), 0.78–0.97 (m, 12H), 1.11 (p, J = 7.0 Hz, 12H), 2.06 (m, 16H), 3.73 (p, J = 6.8 Hz, 2H), 7.34 (m, 12H), 7.67 (s, 4H), 7.73–7.77 (m, 8H), 7.80–7.84 (m, 6H), 8.35 (d, J = 1.5 Hz, 2H). 13C NMR (CDCl3, 300 MHz), δ (ppm): 13.47, 13.87, 23.04, 23.14, 26.05, 40.40, 55.19, 117.61, 119.57, 119.67, 119.77, 119.99, 121.51, 122.84, 122.92, 123.53, 126.17, 126.79, 126.85, 126.88, 127.22, 127.88, 128.24, 128.53, 134.11, 139.23, 139.95, 140.51, 140.60, 140.75, 141.01, 150.77, 150.90, 151.02, 151.56. MALDI-TOF: m/z 1300.9 (M+). Anal. Calcd for C98H109N: C 90.48, H 8.45, N 1.08; found: C 90.38, H 8.38, N 1.15.
9-Butyl-1,3,6,8-tetrakis-(9,9-dibutyl-9H-fluoren-2-yl)-9H-carbazole (12).
12 was prepared by a similar method to 1.White solid. Yield: 45%. mp 144.2 °C. 1H NMR (CDCl3, 500 MHz), δ (ppm): 0.32–0.44 (m, 10H), 0.46–0.55 (m, 3H), 0.73 (t, J = 7.0 Hz, 24H), 0.79–0.90 (m, 10H), 1.00–1.06 (m, 4H), 1.11 (p, J = 7.0 Hz, 12H), 2.07 (m, 16H), 3.72 (s, 2H), 7.30–7.38 (m, 12H), 7.69–7.71 (m, 4H), 7.74–7.77 (m, 8H), 7.83 (d, J = 8.0 Hz, 6H), 8.35 (s, 2H). 13C NMR (CDCl3, 500 MHz), δ (ppm): 13.53, 13.83, 19.41, 23.11, 23.14, 26.10, 40.38, 46.11, 55.23, 117.59, 119.66, 119.77, 119.98, 121.49, 122.92, 122.94, 123.53, 126.18, 126.80, 126.86, 127.19, 128.40, 128.56, 134.28, 139.32, 139.98, 140.58, 140.82, 141.06, 150.82, 150.93, 151.15, 151.58. FAB MS: m/z 1328 (M+). Anal. Calcd for C100H113N: C 90.38, H 8.57, N 1.05; found: C 90.22, H 8.76, N 1.02.
4.3 Device fabrication and measurements
The OLED devices were fabricated in the same run by vacuum deposition at a base pressure of 4 × 10−4 Pa in one pump-down. Before the OLEDs fabrication, commercial ITO coated glass with sheet resistance of 10 Ω/□ used as the starting substrates were cleaned by routine cleaning process and oxygen plasma treatment. Organic chemical used for fabricating devices were sublimed twice prior to use. The OLEDs were fabricated in the following configuration: ITO on glass substrate (anode), 5 nm of MoO3, 30 nm of the hole-transport and emitting layer made of compounds 1, 4 and 10, 10 nm of TPBI, 40 nm of Alq3, 1 nm of LiF and 100 nm of Al (cathode). Additionally, the MoO3, TPBI, Alq3, and LiF were functioned as hole injection, exciton confinement, electron transport, and electron injection, respectively. The growth rates, which are controlled by the quartz thickness monitor, were 0.1, 0.01, and 1 nm s−1 for organics, inorganics, and Al electrode, respectively. The active area of the device, defined by the overlap of the ITO and the Al electrode was about 0.08 cm2. The devices were tested at room temperature under ambient conditions with no protective encapsulation. The EL characteristics of the devices were measured using a fiber spectrometer (Ocean-Optics USB 2000). The current density (J)-luminance (L)-voltage (V) characteristics of the devices was measured using a Keithley SMU 2400 source-measure unit and a BM-8 multi-function radiant measurement meter.
Acknowledgements
This work was supported by the National Natural Science Foundation of China (Grant No. 20972097), the Major Program in Key Field of Guangdong Province Government (Grant No. 2003-03), R & D funds for Basic Research Program of Shenzhen (Grant No. 201120). We acknowledge the Zhou Xiang group for their supporting with OLED fabrication and test system in Sun-Yat Sen University.
References
- T. Fuhrmann and J. Salbeck, MRS Bull., 2003, 28, 354 CrossRef CAS.
- A. C. Grimsdale, K. L. Chan, R. E. Martin, P. G. Jokisz and A. B. Holmes, Chem. Rev., 2009, 109, 897 CrossRef CAS.
- M. A. Baldo, M. E. Thompson and S. R. Forrest, Nature, 2000, 403, 750 CrossRef CAS.
- S. Reineke, F. Lindner, G. Schwartz, N. Seidler, K. Walzer, B. Lüssem and K. Leo, Nature, 2009, 459, 234 CrossRef CAS.
- C. W. Tang and S. A. Van Slyke, Appl. Phys. Lett., 1987, 51, 913 CrossRef CAS.
- A. Mikami, Y. Mizuno and S. Takeda, Dig. Tech. Pap. - Soc. Inf. Disp. Int. Symp., 2008, 39, 215 CrossRef.
- C. G. Zhen, Z. K. Chen, Q. D. Liu, Y. F. Dai, R. Y. C. Shin, S. Y. Chang and J. Kieffer, Adv. Mater., 2009, 21, 2425 CrossRef CAS.
- Y. Yu, M. Nakano and T. Ikeda, Nature, 2003, 425, 145 CrossRef CAS.
- F. Memarzadeh, R. N. Olmsted and J. M. Bartley, Am. J. Infect. Control, 2010, 38, 13 CrossRef.
- O. Lehmann and M. Stuke, Science, 1995, 270, 1644 CAS.
- A. Y. Bobrovsky, N. I. Boiko and V. P. Shiaev, Adv. Mater., 1999, 11, 1025 CrossRef CAS.
- O. Pieroni, A. Fissi, N. Angelini and F. Lenci, Acc. Chem. Res., 2001, 34, 9 CrossRef CAS.
- L. R. Dalton, J. Phys.: Condens. Matter, 2003, 15, 897 CrossRef.
- M. Berggren, M. Granström, O. Inganäs and M. Andersson, Adv. Mater., 1995, 7, 900 CrossRef CAS.
- L. Zou, V. Savvate'ev, J. Booher, C. H. Kim and J. Shinar, Appl. Phys. Lett., 2001, 79, 2282 CrossRef CAS.
- K. Okumoto and Y. Shirota, Appl. Phys. Lett., 2001, 79, 1231 CrossRef CAS.
- S. Hoshino, K. Furukawa, K. Ebata, C. H. Yuan and H. Suzuki, J. Appl. Phys., 2000, 88, 2892 CrossRef CAS.
- T. C. Chao, Y. T. Lin, C. Y. Yang, T. S. Hung, H. C. Chou, C. C. Wu and K. T. Wong, Adv. Mater., 2005, 17, 992 CrossRef CAS.
- P. Schrögel, A. Tomkevičienė, P. Strohriegl, S. T. Hoffmann, A. Köhler and C. Lennartz, J. Mater. Chem., 2011, 21, 2266 RSC.
- R. M. Adhikari, R. Mondal, B. K. Shah and D. C. Neckers, J. Org. Chem., 2007, 72, 4727 CrossRef CAS.
- N. Blouin and M. Leclerc, Acc. Chem. Res., 2008, 41, 1110 CrossRef CAS.
- Z. J. Zhao, J. H. Li, X. P. Chen, P. Lu and Y. Yang, Org. Lett., 2008, 10, 3041 CrossRef CAS.
- P. L. T. Boudreault, N. Blouin and M. Leclerc, Adv. Polym. Sci., 2008, 212, 99 CAS.
- R. M. Adhikari, D. C. Neckers and B. K. Shah, J. Org. Chem., 2009, 74, 3341 CrossRef CAS.
- S. H. Ye, Y. Q. Liu, J. M. Chen, K. Lu, W. P. Wu, C. Y. Du, Y. Liu, T. Wu, Z. G. Shuai and G. Yu, Adv. Mater., 2010, 22, 4167 CrossRef CAS.
- W. Jiang, L. Duan, J. Qiao, G. Dong, D. Zhang, L. Wang and Y. Qiu, J. Mater. Chem., 2011, 21, 4918 RSC.
- K. Brunner, A. V. Dijken, H. Börner, J. J. A. M. Bastiaansen, N. M. M. Kiggen and B. M. W. Langeveld, J. Am. Chem. Soc., 2004, 126, 6035 CrossRef CAS.
- J. Y. Shen, X. L. Yang, T. H. Huang, J. T. Lin, T. H. Ke, L. Y. Chen, C. C. Wu and M. C. P. Yeh, Adv. Funct. Mater., 2007, 17, 983 CrossRef CAS.
- S. Rashidnadimi, T. H. Hung, K. T. Wong and A. J. Bard, J. Am. Chem. Soc., 2008, 130, 634 CrossRef CAS.
- W. Y. Hung, L. C. Chi, W. J. Chen, Y. M. Chen, S. H. Chou and K. T. Wong, J. Mater. Chem., 2010, 20, 10113 RSC.
- Y. Shirota, J. Mater. Chem., 2000, 10, 1 RSC.
- Y. W. Liu, F. F. Niu, J. R. Lian, P. J. Zeng and H. B. Niu, Synth. Met., 2010, 160, 2055 CrossRef CAS.
- F. Cherioux and L. Guyard, Adv. Funct. Mater., 2001, 11, 305 CrossRef CAS.
- S. A. Ponomarenko, S. Kirchmeyer, A. Elschner, B. H. Huisman, A. Karbach and D. Drechsler, Adv. Funct. Mater., 2003, 13, 591 CrossRef CAS.
- R. D. Bettignies, Y. Nicolas, P. Blanchard, E. Levillain, J. M. Nunzi and J. Roncali, Adv. Mater., 2003, 15, 1939 CrossRef.
- L. Duan, L. D. Hou, T. W. Lee, J. Qiao, D. Q. Zhang, G. F. Dong, L. D. Wang and Y. Qiu, J. Mater. Chem., 2010, 20, 6392 RSC.
- S. Tang, M. R. Liu, P. Lu, H. Xia, M. Li, Z. Q. Xie, F. Z. Shen, C. Gu, H. P. Wang, B. Yang and Y. G. Ma, Adv. Funct. Mater., 2007, 17, 2869 CrossRef CAS.
- K. R. J. Thomas, J. T. Lin, Y. T. Tao and C. W. Ko, J. Am. Chem. Soc., 2001, 123, 9404 CrossRef CAS.
- Z. X. Wang, H. X. Shao, J. C. Ye, L. Zhang and P. Lu, Adv. Funct. Mater., 2007, 17, 253 CrossRef CAS.
- J. N. Moorthy, P. Natarajan, P. Venkatakrishnan, D. F. Huang and T. J. Chow, Org. Lett., 2007, 9, 5215 CrossRef CAS.
- K. Naito and A. Miura, J. Phys. Chem., 1993, 97, 6240 CrossRef CAS.
- K. Naito, Chem. Mater., 1994, 6, 2343 CrossRef CAS.
- V. Promarak, M. Ichikawa, T. Sudyoadsuk, S. Saengsuwan, S. Jungsuttiwong and T. Keawin, Thin Solid Films, 2008, 516, 2881 CrossRef CAS.
- J. Y. Li, D. Liu, Y. Q. Li, C. S. Lee, H. L. Kwong and S. T. Lee, Chem. Mater., 2005, 17, 1208 CrossRef CAS.
- C. F. Qiu, L. D. Wang, H. Y. Chen, M. Wong and H. S. Kwok, Appl. Phys. Lett., 2001, 79, 2276 CrossRef CAS.
- M. Kinoshita, H. Kita and Y. Shirota, Adv. Funct. Mater., 2002, 12, 780 CrossRef CAS.
- K. Okumoto and Y. Shirota, Chem. Mater., 2003, 15, 699 CrossRef CAS.
- Z. Q. Gao, Z. H. Li, P. F. Xia, M. S. Wong, K. W. Cheah and C. H. Chen, Adv. Funct. Mater., 2007, 17, 3194 CrossRef CAS.
- S. J. Lee, J. S. Park, K. J. Yoon, Y. I. Kim, S. H. Jin, S. K. Kang, Y. S. Gal, S. Kang, J. Y. Lee, J. W. Kang, S. H. Lee, H. D. Park and J. J. Kim, Adv. Funct. Mater., 2008, 18, 3922 CrossRef CAS.
- T. X. Li, T. Yamamoto, H. L. Lan and J. J. Kido, Polym. Adv. Technol., 2004, 15, 266 CrossRef CAS.
- Y. J. Pu, M. Higashidate, K. Nakayama and J. Kido, J. Mater. Chem., 2008, 18, 4183 RSC.
- Z. Q. Jiang, Z. Y. Liu, C. L. Yang, C. Zhong, J. G. Qin, G. Yu and Y. Q. Liu, Adv. Funct. Mater., 2009, 19, 3987 CrossRef CAS.
- Q. D. Liu, J. P. Lu, J. F. Ding, M. Day, Y. Tao, P. Barrios, J. Stupak, K. Chan, J. J. Li and Y. Chi, Adv. Funct. Mater., 2007, 17, 1028 CrossRef CAS.
- J. N. Demas and G. A. Crosby, J. Phys. Chem., 1971, 75, 991 CrossRef.
- S. Fukuzumi, H. Kotani, T. Suenobu, S. Hong, Y. M. Lee and W. Nam, Chem.–Eur. J., 2010, 16, 354 CrossRef CAS.
|
This journal is © The Royal Society of Chemistry 2011 |
Click here to see how this site uses Cookies. View our privacy policy here.