DOI:
10.1039/C1RA00333J
(Paper)
RSC Adv., 2011,
1, 1756-1762
Magnetically separable carbon capsules loaded with laccase and their application to dye degradation†
Received
22nd June 2011
, Accepted 2nd September 2011
First published on 25th October 2011
Abstract
A synthetic method to generate uniform sub-micrometric magnetic mesoporous carbon capsules (diameter ∼440 ± 20 nm) made up of ferrite nanoparticles (size ∼18 nm) inserted inside the mesoporous shell (∼50 nm of thickness) is presented. The fabrication procedure consists of two steps: a) the synthesis of carbon capsules by using the porogen agent employed to create silica microspheres as a carbon source, and b) the incorporation of magnetic iron oxide nanoparticles inside the mesoporous shell. Although a large quantity of iron oxide nanoparticles is incorporated (∼30 wt%), the magnetic nanocomposite still retains a large and accessible porosity made up of mesopores in the 2–8 nm range. This magnetic composite was used to immobilize a valuable bulky industrial enzyme (laccase). The large amount of immobilized biomolecules, (∼1000 mg g−1 of support) suggests that a considerable fraction of this substance is accommodated in the hollow core of the capsules. The catalytic activity of the immobilized laccase was demonstrated by the degradation of two anthraquinone dyes (i.e. Acid Green 25 and Remazol Brilliant Blue R). It was found that the immobilized enzyme retains its activity (>90%) even after multiple cycles and also after long storage times. The incorporation of ferrite nanoparticles greatly facilitates the manipulation of the immobilized biocatalyst, since it can be easily and quickly recovered from liquid media by the simple application of an external magnetic field.
1. Introduction
Sub-micrometric hollow particles (capsules) have recently attracted widespread attention due to the fact that they combine a small and uniform size with the unique feature of providing a protected macroporous core suitable for encapsulating a large variety of substances.1,2 Specifically, porous inorganic capsules are of great interest because they combine a high permeability owing to their porous shell with a good chemical inertness and mechanical stability. The incorporation of a variety of functionalities (i.e. magnetic, catalytic, electrochemical, etc.) to these hollow particles enhances their level of usefulness.3,4 In particular, mesoporous carbon capsules are important because they combine the above properties with a good electronic conductivity.5–8 These hollow carbon particles have also shown themselves to be highly efficient as catalytic supports.9–11 In this respect, we recently reported the fabrication of core@shell materials consisting of iron oxide nanoparticles confined within the macroporous core of carbon capsules, surrounded by a mesoporous shell.12 This composite exhibited a high catalytic performance in the heterogeneous Fenton process.13
Mesoporous materials with silica, carbon or polymeric frameworks have been extensively employed as carriers for the manipulation of bulky biomolecules (e.g. separation, adsorption, immobilization or drug-delivery).14–16 In particular, mesoporous hollow microparticles constitute an ideal container for storing large amounts of biomolecules due to their unique structural properties, i.e. a large macroporous core and a mesoporous shell.17,18 In order for these hollow particles to fulfil their role as substrates for immobilizing biomolecules, it is important that they be easily separated from the reaction medium. This can be accomplished by the incorporation of magnetic functionalities. Several examples of the use of magnetic porous hollow silica particles for this purpose can be found in the literature.19–21 A few reports also refer to the role of magnetic mesoporous carbon capsules as carriers for the adsorption of biomolecules.12,22,23 In particular, we have demonstrated the capacity of these magnetic carbon capsules to act as carriers for the immobilization of lysozyme.12,22 In all of these reports, the procedure used for the synthesis of the carbon capsules is complex as it requires several steps and the involvement of foreign substances. Indeed, it entails infiltrating mesoporous silica nanospheres with a monomer (i.e. furfuryl alcohol), which is subsequently polymerized with the aid of a catalyst that has been previously deposited within the silica pores. As an alternative to this multistep conventional procedure, we recently proposed a simpler synthetic route to obtain mesoporous carbon capsules, which employs the organic moiety from the porogen agent used to synthesize silica nanospheres as a unique carbon source.24 This procedure greatly simplifies the fabrication of mesoporous carbon capsules. In the present work, we explore the possibility of using the carbon capsules fabricated by this method as carriers for the manipulation of biomolecules. To facilitate the separation of these particles from the reaction medium, magnetic nanoparticles (i.e.iron oxide spinel) were inserted into the mesoporous shell of the carbon capsules. This magnetic composite was proven in relation to the immobilization of an enzyme (laccase). The following features were investigated: a) the structural characteristics of the magnetic carbon capsules, b) the ability of the composites to immobilize laccase and c) the catalytic activity of this biocatalytic system. Laccase was selected for the immobilization because of its potential technological applications. Indeed, this enzyme is able to catalyze the oxidation of various phenolic and aromatic substrates, and simultaneously reduce molecular oxygen to water.25 This property is of great importance for food, analytical and biosensor technologies, waste detoxification and textile dye transformation.26 In this study, we demonstrate the usefulness of this biocatalyst (laccase immobilized on magnetic carbon capsules) by applying it to degrade two textile dyes, i.e. Acid Green 25 and Remazol Brilliant Blue. Moreover, the catalytic stability and the re-utilization of this biocatalytic system are also examined.
2. Experimental section
2.1 Preparation of materials
The synthesis of carbon capsules with macroporous hollow cores and mesoporous shells was performed according to a procedure recently reported by us.24 Briefly, 1 g of uncalcined sub-micrometre-size solid core/mesoporous shell silica particles, prepared as reported by Büchel et al.,27 was treated with H2O2 (30 wt% in water) at room temperature for 15 h. The oxidised product was collected by centrifugation and redispersed in a mixture of 10 g of H2O and 18 mmol of H2SO4. This mixture was heat treated in air at 100 °C for 5 h and at 160 °C for 15 h. The dark solid obtained was heated under N2 up to 800 °C (3 °C min−1) for 1 h. Finally, the carbonized sample was treated with hydrofluoric acid (40%) in order to dissolve the silica framework. The carbonaceous residue was collected by filtration, washed with distilled water and dried at 120 °C. These carbon capsules were designated as HC.
The incorporation of magnetic iron oxide nanoparticles to the carbon capsules was carried out according to the following methodology: a HC sample was impregnated with a solution of Fe(III) nitrate (Aldrich) dissolved in ethanol (0.2 g iron nitrate/g ethanol) until incipient wetness and dried at 50 °C in a vacuum oven. This process was repeated several times until ∼30 wt% of inorganic phase (Fe3O4) was attained in the composite. After that, the impregnated sample was exposed to propionic acid vapours at 80 °C in a closed vessel for 15 h, the propionic acid acting as a soft reducing agent in accordance with the mechanism proposed by Bourlinos et al.28 Finally, the impregnated sample was thermally treated under N2 at 260 °C for 2 h. The resulting composite was denoted as MHC.
2.2 Materials characterization
Scanning electron microscopy (SEM) images, obtained with a Quanta FEG650 (FEI) instrument, and transmission electron micrographs (TEM), taken on a JEOL (JEM-2000 FXII), were the techniques used to examine the morphology of the samples. Nitrogen adsorption and desorption isotherms were performed at −196 °C in a Micromeritics ASAP 2020 volumetric adsorption system. The BET surface area was calculated from the analysis of the sorption isotherm in the relative pressure range of 0.04–0.20. The total pore volume was calculated from the amount of nitrogen adsorbed at a relative pressure of 0.99. The pore size distribution (PSD) was calculated by means of the Kruk-Jaroniec-Sayari method.29 The primary mesopore volume (Vm) and external surface area (Sext) were estimated using the αs-plot method. The reference adsorption data used for the αs analysis of the carbon samples correspond to a non-graphitized carbon black sample.30X-ray diffraction (XRD) patterns were obtained on a Siemens D5000 instrument operating at 40 kV and 20 mA and using CuKa radiation (λ = 0.15406 nm) source. Diffuse reflectance Fourier-Transform Infrared (FT-IR) spectra were recorded on a Nicolet Magna-IR 560 spectrometer fitted with a diffuse reflection attachment. The thermogravimetric analysis was performed in a CI Electronics system.
2.3
Enzyme immobilization
A MHC magnetic composite was employed to immobilize laccase from Trametes versicolor. The organic reagent (OR) containing the pure enzyme was purchased from Sigma-Aldrich. According to the information of the supplier, the pure enzyme content (Laccase, Lc) in the organic reagent (OR) was 10 wt%. This value was used to calculate the amount of enzyme immobilized. To immobilize the enzyme on the MHC particles, around 10 mg of magnetic nanocomposite was dispersed at room temperature in 10 mL of laccase solution (initial concentration 0.1–2.5 mg OR·mL−1) prepared with 0.025 M sodium acetate buffer (pH 4.2). The adsorption experiments were carried out in closed vessels to avoid any evaporation, with continuous orbital stirring for 45 h. Subsequently, the MHC particles loaded with laccase were separated by centrifugation and the solid was washed several times with a buffer solution until no protein was detected in the supernatant. The laccase-magnetic composite (Lc-MHC) was then stored in a fresh acetate buffer solution at 4 °C before use. To evaluate the amount of immobilized enzyme, the concentration of protein in the solution was periodically monitored by means of spectrophotometric measurements using Coomassie Brilliant Blue purchased from Aldrich.31 A calibration curve was constructed employing several OR solutions with concentrations in the 0.1–2.5 mg mL−1 range. The adsorbed amount of OR/Lc was deduced from the difference between the amount of OR/Lc introduced into the reaction mixture and the amount of OR/Lc found in the supernatant and washing solutions. Moreover, the OR/Lc loaded on the inorganic phase (MHC) was also determined by using TGA to calculate the weight loss resulting from heating the hybrid composite under nitrogen up to 500 °C.
2.4.
Dye
degradation experiments
For the dye degradation experiments, two anthraquinone-type synthetic dyes purchased from Aldrich were selected: a) Acid Green 25 (AG 25) and b) Remazol Brilliant Blue (RBBR) (see Fig. S-1 in the ESI for the chemical characteristics†). These experiments were conducted on magnetic nanocomposites with and without immobilized enzyme. In a typical assay, around 10 mg of support was dispersed in 10 mL of dye solution (initial concentration 0.1–2 mg mL−1 for AG 25, and 0.1–0.3 mg mL−1 for RBBR) in a sodium acetate buffer 0.025 M (pH 4.2) with orbital stirring. The mixture was incubated for a maximum of 25 h in the case of AG25 and 50 h in the case of RBBR. The catalytic activity of the immobilized laccase was evaluated from the percentage of degraded dye, which was periodically monitored. The concentration of the dye in the solution was measured with an UV-vis spectrophotometer (Shimadzu UV-2401PC) at the wavelengths of maximum absorbance 608 nm and 592 nm for AG25 and RBBR respectively. In order to evaluate the extent of degradation, spectra in the 200–700 nm range were collected at different reaction times. To determine whether the Lc-MHC biocatalyst could be reused, recycling experiments were performed with both dyes. At the end of each cycle, the catalyst was washed three times with a buffer solution (3 × 10 mL).
3. Results and discussion
3.1. Structural properties of carbon capsules and magnetic nanocomposites
The structural characteristics of the carbon capsules and the magnetic composites were investigated by SEM, TEM, X-ray diffraction and gas adsorption analysis. The SEM and TEM images for both the carbon capsules and magnetic nanocomposites are shown in Fig. 1.
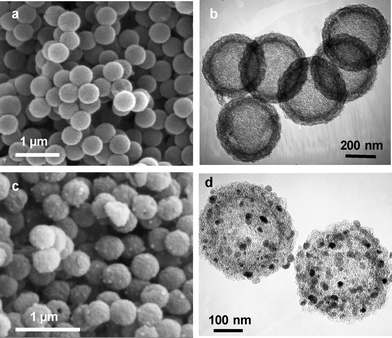 |
| Fig. 1
SEM images (a, c) and TEM images (b, d) of mesoporous carbon capsules, HC (a and b) and magnetic carbon capsules, MHC (c and d). | |
The SEM images in Fig. 1a and c reveal that both materials have an identical external appearance and are composed of sub-micrometric particles with a spherical morphology and a very uniform diameter of around 440 ± 20 nm. It can also be seen that the morphology of the composites is preserved after the incorporation of inorganic nanoparticles (Fig. 1c). The TEM image in Fig. 1b shows the internal structure of a carbon capsule, which is formed by a porous layer of thickness ∼50 nm and a central macroporous core ∼350 nm in diameter. The SEM microphotograph presented in Fig. 1c reveals the presence of inorganic nanoparticles (white spots) deposited on the outer surface of the carbon capsules. These are clearly visible in the TEM image in Fig. 1d. This image reveals that the inorganic nanoparticles are exclusively deposited on the porous shell and that they only occupy a small fraction of the porosity, the rest of the pores remaining empty. Proof of the formation of the ferrite iron oxide nanoparticles was obtained by X-ray diffraction. Thus, the XRD pattern in Fig. 2a reveals that these particles consist of iron oxide spinel nanomagnets (magnetite@maghemite) and that they have a size of around 18 nm, which was deduced by applying the Scherrer equation to the (311) diffraction peak. In general, the crystallite sizes of the magnetic nanoparticles obtained by XRD are slightly smaller than the diameters deduced by TEM inspection, which suggests that ferrite nanoparticles are formed by the aggregation of two or three crystallites. The room-temperature magnetization curve for the MHC particles was recorded (see Fig. 2b). As can be seen from the figure, this sample displays irreversible magnetic behaviour (coercivity field of 65 Oe as deduced from Fig. 2b/inset) with a saturation magnetization value of 12.8 emu g−1. The magnetic properties of these nanocomposites are exemplified in Fig. 2c, which shows that the MHC particles are easily separated from the liquid media by applying an external magnetic field. This magnetic separation occurs in a relatively short period of time (<2 min).
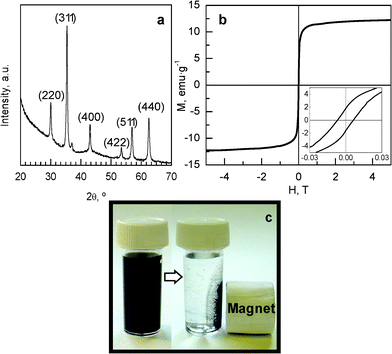 |
| Fig. 2 (a) XRD pattern of the MHC sample. (b) Field-dependent magnetization of MHC particles at room temperature (inset: low-field magnetization). (c) Example of magnetic separation of the MHC capsules. | |
The textural characteristics of the porous carbon capsules and the corresponding magnetic composites were examined by means of nitrogen physisorption (Fig. 3). The textural data are summarized in Table 1. The carbon capsules employed to prepare these composites exhibit a well-developed porosity as is deduced from the nitrogen sorption isotherm in Fig. 3a. A capillary condensation step is observed at p/po ∼0.5–0.6 which evidences the presence of mesopores. These mesopores have a size in the 2–12 nm range (maximum at 4.3 nm) as can be deduced from the pore size distribution shown in Fig. 3b. The carbon capsules have a large BET surface area of 1620 m2 g−1 and a high pore volume of 2.3 cm3 g−1, 1.73 cm3 g−1 corresponding to the shell-confined pores, which is calculated by applying the αs-plot analysis to the adsorption branch. When magnetic nanoparticles are incorporated within the porous shell of the carbon capsules, the resulting magnetic composite, with ∼30 wt% of iron oxide ferrite nanoparticles, experiences a reduction in textural properties due to the incorporation of the inorganic phase and also to the filling or blockage of a number of pores (see Fig. 3a and Table 1). The pore size distribution in Fig. 3b also shows that the insertion of iron oxide nanoparticles leads to a reduction in the number of larger mesopores, which suggests that these pores are preferentially occupied. However a large number of mesopores in the MHC sample still remain unfilled, as can be inferred from the textural properties of this material (see Table 1, Fig. 3b and Fig. 1d). By comparing the pore volumes of the HC and MHC samples, it is deduced that the inorganic nanoparticles occupy ∼35% of the pore volume of the mesoporous shell. Hence, a significant fraction of the porosity of the magnetic composite (MHC) remains unobstructed and available for adsorbing or transporting substances towards and from the inner macroporous core of the capsule.
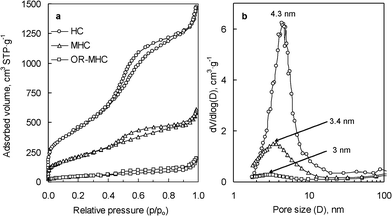 |
| Fig. 3 (a) Nitrogen adsorption isotherms and (b) pore size distributions of the carbon capsules (HC), the magnetic nanocomposite (MHC) and the composite loaded with biomolecules (OR-MHC). | |
Table 1 Textural properties of the hollow carbon (HC) particles, the iron oxide ferrite/carbon capsule composite (MHC) and the composite after adsorption of the organic reagent (OR-MHC)
Sample |
SBET (m2 g−1) |
Vp (cm3 g−1)a |
Pore size (nm)b |
FWHM (nm)c |
α
s-Plot results |
Vm (cm3 g−1)d |
Sext (m2 g−1)e |
Pore volume determined at p/po = 0.99.
Maximum of the pore size distribution.
Full Width at Half Maximum of the pore size distribution.
Pore volume of structural mesopores.
External surface area.
|
HC |
1620 |
2.3 |
4.3 |
2.8 |
1.73 |
168 |
MHC |
780 |
0.94 |
3.4 |
4.7 |
0.78 |
92 |
OR-MHC |
160 |
0.3 |
3.0 |
4.8 |
0.18 |
63 |
3.2. Immobilization of laccase over magnetically separable carbon capsules
To demonstrate the potential of the magnetic hollow carbon particles to manipulate bulky biomolecules, the immobilization and the catalytic activity of the enzyme laccase was investigated. This enzyme is a cuproprotein (molecular dimensions of 6.5 × 5.5 × 4.5 nm3 and a molecular weight of 68 kDa32) capable of catalyzing the oxidation of various aromatic compounds.25 In our experiments, we used a variety of yellow laccase formed by binding the aromatic products of lignin degradation with blue laccase. These aromatic substances can act as exogenous mediators by increasing the capacity of laccase to oxidize non-phenolic compounds.33,34 Taking into account the fact that the active enzyme in the organic reagent (OR) is 10 wt%, two values were derived from adsorption experiments: i) the amount of adsorbed organic reagent (OR) and ii) the amount of immobilized laccase (Lc). The immobilization experiments were carried out over a wide range of concentrations of OR adsorbate (between 0.1 and 2.5 mg mL−1), in an acetate buffer solution with a pH of 4.2, which is the isoelectric point of the enzyme.35 At this point (pH∼pI), the maximum of enzyme loading is attained.36,37 As a consequence of the adsorption of biomolecules on the magnetic composite, the hybrid organic–inorganic material (OR-MHC) showed a notable reduction in its textural properties in relation to the MHC sample (see Table 1 and Fig. 3). This result indicates that a large number of pores have been filled by the biomolecules. By comparing the mesopore volumes measured for MHC (0.78 cm3 g−1) and for OR-MHC (0.18 cm3 g−1) and the amount of OR adsorbed, it can be deduced that >50% of the organic reagent has entered the macroporous core of the carbon capsules. This finding clearly shows that the hollow core acts as a nano-container which considerably enhances the capacity of immobilization beyond what one would expect from the relative small volume of the mesopore shell. TGA was also employed to determine the amount of adsorbed OR and the obtained results (see Fig. S-2 in the ESI†) confirm those achieved by means of the UV-vis technique.
The kinetics of the adsorption of OR on the MHC sample is illustrated in Fig. 4a. It can be seen that the immobilization process is relatively fast, and that the equilibrium of adsorption is achieved in ∼20 h in all cases. Fig. 4b shows the equilibrium adsorption isotherms for the OR/Lc-MHC system at 20 °C and pH 4.2. It can be seen that the maximum amount of OR adsorbed on the magnetic carbon capsules is ∼1000 mg g−1 of support. Taking into account the percentage of pure laccase in the organic reagent (OR), the adsorption isotherm for this enzyme is also represented in Fig. 4b. The adsorption equilibrium data were adjusted by means of the Langmuir model. The solid lines in Fig. 4b correspond to the best fit of the Langmuir equation. This adjustment allows us to estimate the maximum amount of laccase that can be adsorbed (monolayer adsorption capacity) is 140 mg Lc·g−1. In comparison to other mesoporous magnetic supports reported in the literature, the magnetic carbon capsules presented here provide a higher laccase immobilization uptake (see Table S-1 in the ESI†). This large immobilization capacity can be attributed to the macroporous core that acts as a nano-container allowing a higher amount of enzyme to be stored in comparison to other more conventional mesoporous supports.
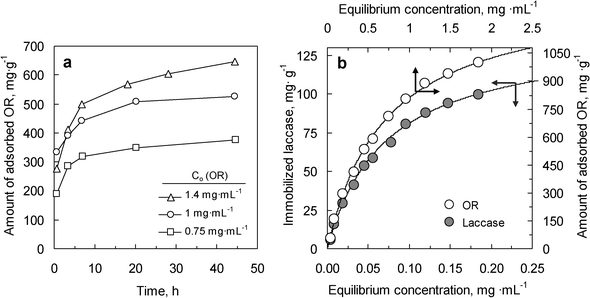 |
| Fig. 4 (a) Variation of the amount of OR adsorbed with time at different initial concentrations of adsorbate. (b) Adsorption isotherms for the OR adsorbate and pure laccase over MHC (20 °C, pH = 4.2). Solid lines in b represent the best fit of experimental data to the Langmuir model. | |
To examine the structure of the immobilized laccase, FT-IR analysis was conducted on the pure laccase and Lc-MHC system (Fig. 5). Table S-2 (ESI†) shows the IR frequencies associated to the functional groups of proteins.38
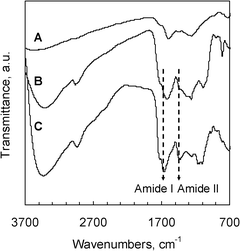 |
| Fig. 5
FT-IR spectra of (A) magnetic composite (MHC), (B) laccase immobilized over magnetic carbon capsules (Lc-MHC), and (C) free laccase. | |
Specifically, the amide I and II infrared bands provide detailed information about the secondary structure of the proteins. Thus, the amide I band (∼1650 cm−1) is ascribed to the C
O stretching vibration of the peptide linkages, whereas the amide II band (∼1500 cm−1) is attributed to the bending and stretching mode of the N–H and C–N vibrations, respectively.39 The free laccase exhibits the characteristic IR bands of proteins. In the case of the adsorbed laccase (Lc-MHC sample), it can be observed that the amide I band and the amide II band appear at around 1650 cm−1 and 1450 cm−1 respectively. The rest of the protein bands at 3450, 2955, 1290 and 815 cm−1 also appear in the IR spectrum of the Lc-MHC sample. These results show that the secondary structure of the laccase remains unchanged, indicating that the intrinsic properties of the adsorbed enzyme (e.g.catalytic activity) are preserved.
3.3.
Degradation of dyes by immobilized laccase and reusability
In order to assess the usefulness of the Lc-MHC sample as a biocatalyst, the catalytic potential of this system must be confirmed. Accordingly, we analyzed the enzymatic activity of the Lc-MHC sample in relation to the degradation of textile dyes, which are usually toxic and recalcitrant due to their fused aromatic structure. In this context, we selected two anthraquinone-type dyes (Acid Green 25 (AG 25) and Remazol Brilliant Blue (RBBR)). The results obtained for the biocatalytic degradation of these dyes by the Lc-MHC system are shown in Fig. 6 and 7. Fig. 6 illustrates the kinetics of the degradation process. It can be observed that for both dyes, the Lc-MHC sample exhibits high rates of degradation, with ∼80% of the dyes being decomposed in ∼1 h. In order to independently assess the ability of immobilized laccase to degrade dyes, we used the DC parameter (Degradation Capacity). This is defined as the amount of dye that is degraded per mass unit of enzyme after 30 min. In the case of the immobilized laccase (Lc-MHC), the DC values calculated for each dye are: 7.3 μmol AG25 mg−1Lc and 9 μmol RBBR mg−1Lc. Obviously, these values are lower than those measured for the free enzyme (i.e. 16 μmol AG25 mg−1Lc and 12.8 μmol RBBR mg−1Lc). However, it must be pointed out that, unlike immobilized laccase, the free enzyme is difficult to recover for reutilization purposes. As an example, the degradation of the AG25 dye is illustrated in Fig. S-3 (ESI†). It is worth mentioning that the degradation of the dyes by the Lc-MHC biocatalyst does not require any external mediator which is remarkably advantageous. For practical purposes however, it is important that the biocatalyst system (Lc-MHC) possess not only a high catalytic activity, but also that this activity be preserved even after multiple cycles. In order to determine whether this is the case, we examined the degradation capacity of the immobilized laccase over at least 6 cycles for both dyes (Fig. 7a and 7b). In both cases, after six consecutive cycles, only a small reduction in degradation activity was observed (i.e. ∼3% for AG25 and ∼7% for RBBR). In the case of the AG25 dye after eight cycles a loss of only ∼5% of activity was detected.
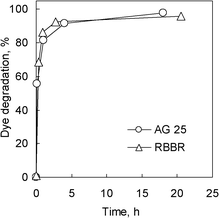 |
| Fig. 6
Dye
degradation profiles over the Lc-MHC biocatalyst as a function of time. | |
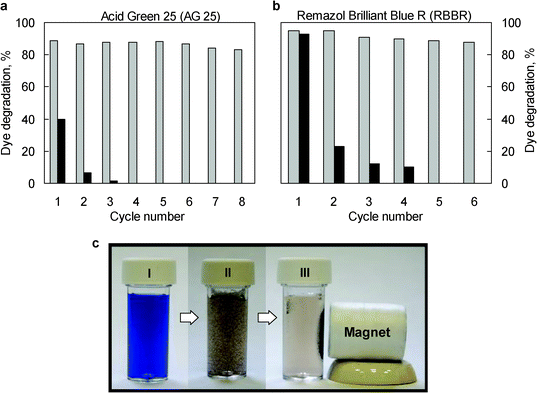 |
| Fig. 7 Reusability of laccase immobilized over magnetic carbon capsules in relation to the degradation of: a) AG 25 and b) RBBR (Reaction time: 2 h). Grey columns correspond to catalytic degradation by means of Lc-MHC, and the black ones to the adsorption of dye on the MHC magnetic composite. c) Image of the sequence of a RBBR degradation reaction: (I) RBBR solution, (II) after addition of Lc-MHC particles and (III) magnetic separation of Lc-MHC particles. | |
Moreover, the activity of the immobilized laccase remained stable after long storage times and after two months the loss of activity was <10% (see Fig. S-3 in the ESI†). These results indicate that the laccase immobilized on magnetic supports exhibits excellent reusability and that the activity of the immobilized enzyme is preserved even after long storage times. Fig. 7a and b show the percentages of dye removed via adsorption by the MHC support over several cycles. It can be seen that the adsorption process is considerably less effective than the biocatalytic degradation by means of Lc-MHC. What is more, the adsorption capacity of the magnetic support disappears after 3 or 4 cycles. These results demonstrate that Lc-MHC is much more effective in eliminating dyes than the MHC sample. Another advantage of the Lc-MHC biocatalyst is that it can be magnetically separated. Thus, Fig. 7 illustrates a typical dye degradation experiment by means of the Lc-MHC system and its separation. It can be seen that once the dye degradation has taken place, the catalytic particles are easily and quickly (<2 min) recovered from the reaction medium by applying an external magnet.
4. Conclusions
In summary, we have illustrated a simple synthetic procedure for fabricating magnetic carbon capsules (diameter ∼440 nm) made up of a macroporous core surrounded by a mesoporous shell (thickness ∼50 nm), containing iron oxide spinel nanoparticles inside the pores. In spite of the presence of the inorganic phase, a large fraction of the porosity of the composite remains unfilled. The unique structure of the magnetic hollow carbon particles allows the immobilization of a large amount of laccase (100 mg g−1 of support), which is introduced into the mesopores of the shell and the macroporous core. The magnetic nanocomposite loaded with laccase was successfully applied to degrade two dyes: Acid Green 25 and Remazol Brilliant Blue R. The Lc-MHC biocatalyst exhibited a good performance in the degradation of both dyes (80% of the dyes were degraded in ∼1 h). Moreover, it was found that the catalytic activity of the Lc-MHC system was preserved even after several reaction cycles (∼95% of the initial activity was retained after eight cycles in the case of the RB25 dye) and that it also retained most of its activity after long storage times (activity loss: <10% after two months). Finally, it is worth noting that this magnetic biocatalyst can be easily and quickly recovered from a liquid medium by means of an external magnetic field, which favours its application.
Acknowledgements
The financial support for this research provided by the MEC (MAT2008-00407) is gratefully acknowledged. P. V.-V. acknowledges the assistance of the Spanish MCyT for the award of a JAE-Predoc grant.
References
- Y. Ma and L. Qi, J. Colloid Interface Sci., 2009, 335, 1 CrossRef CAS.
- X. W. Lou, L. A. Archer and Z. Yang, Adv. Mater., 2008, 20, 3987 CrossRef CAS.
- J. Liu, F. Liu, K. Gao, J. Wu and D. Xue, J. Mater. Chem., 2009, 19, 6073 RSC.
- F. Caruso, Adv. Mater., 2001, 13, 11 CrossRef CAS.
- M. Kim, S. B. Yoon, K. Sohn, J. Y. Kim, C. H. Shin, T. Hyeon and J. S. Yu, Microporous Mesoporous Mater., 2003, 63, 1 CrossRef CAS.
- S. B. Yoon, K. Sohn, J. Y. Kim, C. H. Shin, J. S. Yu and T. Hyeon, Adv. Mater., 2002, 14, 19 CrossRef CAS.
- S. Ikeda, K. Tachi, Y. H. Ng, Y. Ikoma, T. Sakata, H. Mori, T. Harada and M. Matsumura, Chem. Mater., 2007, 19, 4335 CrossRef CAS.
- J. Jang, X. L. Li and J. H. Oh, Chem. Commun., 2004, 794 RSC.
- G. S. Chai, S. B. Yoon, J. H. Kim and J. S. Yu, Chem. Commun., 2004, 2766 RSC.
- M. Kim, K. Sohn, H. B. Na and T. Hyeon, Nano Lett., 2002, 2, 1383 CrossRef CAS.
- S. Ikeda, S. Ishino, T. Harada, N. Okamoto, T. Sakata, H. Mori, S. Kuwabata, T. Torimoto and M. Matsumura, Angew. Chem., Int. Ed., 2006, 45, 7063 CrossRef CAS.
- A. B. Fuertes, M. Sevilla, T. Valdes-Solis and P. Tartaj, Chem. Mater., 2007, 19, 5418 CrossRef CAS.
- T. Valdes-Solis, P. Valle-Vigon, M. Sevilla and A. B. Fuertes, J. Catal., 2007, 251, 239 CrossRef CAS.
- M. Hartmann and D. Jung, J. Mater. Chem., 2010, 20, 844 RSC.
- M. Manzano and M. Vallet-Regi, J. Mater. Chem., 2010, 20, 5593 RSC.
- H. H. P. Yiu and P. A. Wright, J. Mater. Chem., 2005, 15, 3690 RSC.
- Y. Wang and F. Caruso, Chem. Mater., 2005, 17, 953 CrossRef CAS.
- Y. Zhao, L. N. Lin, Y. Lu, S. F. Chen, L. Dong and S. H. Yu, Adv. Mater., 2010, 22, 5255 CrossRef CAS.
- J. Kim, J. E. Lee, J. Lee, J. H. Yu, B. C. Kim, K. An, Y. Hwang, C. H. Shin, J. G. Park, J. Kim and T. Hyeon, J. Am. Chem. Soc., 2005, 128, 688 CrossRef.
- W. Zhao, H. Chen, Y. Li, L. Li, M. Lang and J. Shi, Adv. Funct. Mater., 2008, 18, 2780 CrossRef CAS.
- Y. Zhu, E. Kockrick, T. Ikoma, N. Hanagata and S. Kaskel, Chem. Mater., 2009, 21, 2547 CrossRef CAS.
- A. B. Fuertes, T. Valdes-Solis, M. Sevilla and P. Tartaj, J. Phys. Chem. C, 2008, 112, 3648 CAS.
- L. Guo, X. Cui, Y. Li, Q. He, L. Zhang, W. Bu and J. Shi, Chem.–Asian J., 2009, 4, 1480 CrossRef CAS.
- P. Valle-Vigón, M. Sevilla and A. B. Fuertes, Chem. Mater., 2010, 22, 2526 CrossRef.
- V. Madhavi and S. S. Lele, BioResources, 2009, 4, 1694 Search PubMed.
- S. Witayakran and A. Ragauskas, Adv. Synth. Catal., 2009, 351, 1187 CrossRef CAS.
- G. Büchel, K. K. Unger, A. Matsumoto and K. Tsutsumi, Adv. Mater., 1998, 10, 1036 CrossRef.
- A. Bourlinos, A. Simopoulos, D. Petridis, H. Okumura and G. Hadjipanayis, Adv. Mater., 2001, 13, 289 CrossRef CAS.
- M. Kruk, M. Jaroniec and A. Sayari, Langmuir, 1997, 13, 6267 CrossRef CAS.
- M. Kruk, M. Jaroniec and K. P. Gadkaree, J. Colloid Interface Sci., 1997, 192, 250 CrossRef CAS.
- M. E. Corman, N. Oezturk, N. Bereli, S. Akgol and A. Denizli, J. Mol. Catal. B: Enzym., 2010, 63, 102 CrossRef CAS.
- K. Piontek, M. Antorini and T. Choinowski, J. Biol. Chem., 2002, 277, 37663 CrossRef CAS.
- A. A. Leontievsky, T. Vares, P. Lankinen, J. K. Shergill, N. N. Pozdnyakova, N. M. Myasoedova, N. Kalkkinen, L. A. Golovleva, R. Cammack, C. F. Thurston and A. Hatakka, FEMS Microbiol. Lett., 1997, 156, 9 CrossRef CAS.
- N. N. Pozdnyakova, J. Rodakiewicz-Nowak and O. V. Turkovskaya, J. Mol. Catal. B: Enzym., 2004, 30, 19 CrossRef CAS.
- G. Bayramoglu and M. Y. Arica, Mater. Sci. Eng., C, 2009, 29, 1990 CrossRef CAS.
- A. Vinu, V. Murugesan and M. Hartmann, J. Phys. Chem. B, 2004, 108, 7323 CrossRef CAS.
- T. Valdés-Solís, A. F. Rebolledo, M. Sevilla, A. B. Fuertes, P. Valle-Vigón, O. Bomati-Miguel and P. Tartaj, Chem. Mater., 2009, 21, 1806 CrossRef.
- J. Kong and S. Yu, Acta Biochim. Biophys. Sin., 2007, 39, 549 CrossRef CAS.
- A. Barth, Biochim. Biophys. Acta, Bioenerg., 2007, 1767, 1073 CrossRef CAS.
Footnote |
† Electronic supplementary information (ESI) available. See DOI: 10.1039/c1ra00333j |
|
This journal is © The Royal Society of Chemistry 2011 |
Click here to see how this site uses Cookies. View our privacy policy here.