DOI:
10.1039/C1SC00109D
(Edge Article)
Chem. Sci., 2011,
2, 1356-1361
Metal-mediated fabrication of new functional G-quartet-based supramolecular nanostructure and potential application as controlled drug release system†
Received
24th February 2011
, Accepted 9th April 2011
First published on 12th May 2011
Abstract
The creation and expansion of addressable supramolecular architectures in a facile and economic way with further functions still remain a big challenge in the field of self-assembly. Herein, we demonstrated a novel strategy to construct a metal-mediated G-quartet-based supramolecular nanostructure with controllable functions. By using 5′-GMP as basic building blocks in dilute solution, the Sr2+ promoted assemblies are simple and intuitive, yet cost-effective. The resulting structures can be regarded as completely homogeneous from the structural point of view and the nanowires are much longer than those previously reported. The nanowires can be controlled by chemical input signals, namely, divalent metal ions and a chelating agent. Furthermore, the encapsulating feature of this system towards G-quadruplex-specific ligands might be of particular biological significance, in the framework of nucleic telomerase.
Introduction
Self-assembly of biological important small molecules into nanostructures has evolved as an attractive strategy for fabricating materials with a broad range of potential applications in catalysis, sensing, electronics, medical diagnostics and drug delivery.1–5 Due to the potential non-covalent interactions, nucleobases have been proposed as basic units of supramolecular motifs. Promising candidates for such exploration are guanine and its derivatives. For example, the G-quartet, a motif found in nucleic acid assemblies which is typically formed by cation-templated self-assembly of four guanosine (G) residues,6 has been demonstrated to serve as a powerful scaffold for building supramolecular architectures such as transmembrane ion channels, gelators, hydrogels, thermosensitive materials, soft-organized surfaces, and prototypes of chemical dynamic devices.7–20 In particular, guanosine 5′ monophosphate (5′-GMP) has the distinctive ability to self-associate spontaneously forming ordered helical structures.21 The number of stacked G-quartets depends on the species present and their concentration and can reach relatively high values. For example, Wu et al. have reported the association of 5′-GMP at concentrations between 0.4–0.8 M in an aqueous solutions containing up to 0.5 M Na+. These nanoscale 5′-GMP aggregates are significantly larger than was believed for many years: the average lengths of the columns are between 8 and 30 nm, corresponding to a cylinder composed of 24–87 stacked G-quartets.22 Moreover, Wu et al. also demonstrated the structural details for the 5′-GMP helix in the presence of Na+ ions, the residing of mixed Sr2+ and Na+ ions inside a G-quadruplex channel and the selective binding of monovalent cations to the stacking G-quartet structure.23–25 Very recently, high ordered rod-like structure were constructed only on the surface at mol concentration of 5′-GMP and NaCl26 Although promising in fabricating high-order architectures, these systems have been limited by the critical assembly condition, including the use of high concentration of 5′-GMP and promoting monovalent salts, and/or the need of non-aqueous media. The high salt content in the system hampered the quality of the supramolecular structures and the length of the cylinders obtained in aqueous solution is still relatively small. Furthermore, the thus prepared supramolecular nanostructures are limited for further applications. Therefore, the creation and expansion of addressable supramolecular architectures in a facile and economic way with further functions still remains a big challenge in this field. Herein, we describe the design and construction of metal-mediated G-quartet-based supramolecular nanostructures in dilute solution with controllable functions. To the best of our knowledge, this is the first fine supramolecular architectures are fabricated with low concentrations of unmodified 5′-GMP and promoting cations, as well as a novel example of a G-quadruplex controlled specific drug release system by using G-quartet-based supramolecular nanoassembly.
Results and discussion
Design of Sr-promoted 5′-GMP assembly
The columnar G-quadruplex unit is formed by the vertical stacking of G-quartets. The role of cation templating is to stabilize the unit by coordination to the eight carbonyl oxygen atoms of two sandwiched G-quartets. Recently, it was found that the nature of the cations also controlled the solution properties of these G-quadruplexes. For example, Sr2+, Ca2+ and Mg2+ were found to induce G-wire formation by G-rich sequences such as d(G4T2G4) and telomere DNAs.27–29G-quadruplexes containing divalent cations such as Ba2+ or Sr2+ were recently demonstrated to be thermodynamically and kinetically more stable than G-quadruplexes that contained monovalent Na+ or K+.27,30 In the case of Sr2+, the quadruplex stability was enhanced with some melting temperatures surpassing 95 °C27 and different transition changes were induced.31 The enhanced stability was attributed to the stronger ion–dipole interactions between the cations and the nucleobase oxygens, as well as to a strengthening of the hydrogen bonds of the G-quartet. On the other hand, it has been well established that the mode of ion binding in 5′-GMP aggregates shares great similarity to that found in G-rich DNA sequences.32,33
Our strategy was inspired by the essential role of Sr2+ and the concept is illustrated in Scheme 1. Upon addition of Sr2+, the 5′-GMP assembled into a supramolecular architecture. On the other hand, Na2EDTA induced a reversible structural transition from the high-order structure to monomeric GMP. Therefore, controlled and addressable drug release could be realized by incorporating small ligands into this system. To demonstrate the design principle of this approach, N-methyl mesoprophrin IX (NMM) was first used as a fluorescent probe to demonstrate the presence of G-quartet-based supramolecular architectures. NMM is a commercially available unsymmetrical anionic porphyrin characterized by a pronounced structural selectivity for G-quadruplexes but not for duplex, triplex or single-stranded forms.34 The unique feature of fluorescence enhancement (greater than 20-fold) that occurred only with G-quardruplex DNA has been successfully utilized recently for application in nuclease and DNA detection.35–37 As shown in Fig. 1, 2 μM NMM was added to 20 mM Na2(5′-GMP) before the assembly process occurred and nearly no fluorescence can be seen. In contrast, a substantial fluorescent increase was observed upon the addition of 8 mM SrCl2 to the mixture. This is in great contrast to the case of adding 1 M MCl (M = Li, Na, K), in which nearly no fluorescence was observed (Fig. S1a, ESI†). Notably, substantial fluorescence enhancement was observed when the concentration of 5′-GMP was raised to 100 mM using either 8 mM SrCl2 or 1 M KCl (Fig. S1b, ESI†), which indicated that only 8 mM SrCl2 was sufficient for promoting 5′-GMP assembly and mimics the effect of 1 M K+ solution. Importantly, the white precipitate resulting from the addition of 8 mM SrCl2 to the mixture of 2 μM NMM and 20 mM or 100 mM 5′-GMP at ambient temperature displays strong red emission upon excitation at 399 nm. Since Sr2+ has also been reported to interact with phosphate groups of 5′-GMP as well as to associate with the eight O6 atoms of neighboring guanine bases,38,39 control experiments were carried out to elucidate the Sr-mediated quartet-based supramolecular assembly rather than the Sr-phosphate-based precipitation. No precipitation was observed in the presence of 5′-AMP, UMP and IMP (Fig. S2, ESI†), which ruled out the possibility that the precipitate might result from the interaction between Sr2+ and phosphate groups. Furthermore, among the four nucleotides used, only 5′-GMP gives significant fluorescence enhancement of NMM upon the addition of 8 mM SrCl2 (Fig. 1). This result indicates that the precipitate and fluorescence enhancement process is base specific with the phosphate group playing a limited role. To further demonstrate the Sr2+ promoted G-quartet supramolecular assembly process, 5′-IMP was tested in the same condition. Earlier reports have shown that the 2-amino group of guanine is engaged in base pairing and is essential for the formation of G-structures.40 The only difference between 5′-GMP and 5′-IMP is that the 2-amino group has been deleted in 5′-IMP. Therefore, the absence of fluorescence enhancement in the case of 5′-IMP (Fig. 1) clearly confirmed the vital role of the N2 group of guanine in the Sr2+-promoted G-quartet-based architecture.
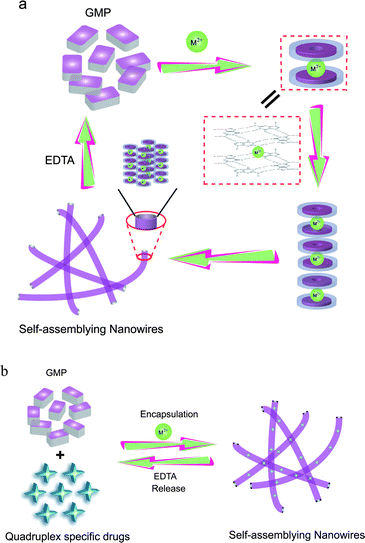 |
| Scheme 1 (a) Proposed model for Sr2+-mediated assembly/disassembly of 5′-GMP into functional nanowires and (b) its application as a controlled G-quadruplex specific drug release system. | |
Characterization of Sr2+/5′-GMP assembly
To gain further support for the proposed design and construction, direct observation of the supramolecular architectures was performed by transmission and scanning electron microscopy (TEM and SEM). The assemblies grow rapidly in aqueous solution, as they are separated from the dispersions as fibrous microstructures. The formation of large nanowires after the addition of 8 mM SrCl2 is shown in Fig. 2a and 2b. Interestingly, the nanowires are relatively uniform with diameters of 50–90 nm and lengths up to micrometres (Fig. S3a, ESI†). The observed width of nanowires is much larger than the size of a single stack G-quartet (2.4–2.6 nm), indicating that the nanowires contain bundled G-quadruplex complexes with Na+ neutralizing the accumulated negative charges of the stack and holding the individual stacks together, mostly by coordination or electrostatic interaction with the phosphate groups on adjacent G-quartet stacks (Fig. S4, ESI†).41 This is in accordance with the previous results that Na+ shows great binding selectivity for surface site25 and Sr2+ ions are known to locate in every other guanine tetrad plane, sitting on the fourfold axis and associating with the eight O6 atoms of neighboring guanine bases in bipyramidal-antiprismatic geometry.38,39 Solid-state 23Na NMR MAS spectra also confirmed this (Table S1 and Fig. S5, ESI†). In addition, X-ray diffraction (XRD) analysis indicated that the nanowires, which were prepared either in the presence or absence of NMM, are amorphous (Fig. S6, ESI†). Furthermore, the fluorescence microscopy image of the 5′-GMP/NMM/Sr2+ assembly also showed strong fluorescence response (Fig. 2c), indicating that these nanowires might have potential application in bioimaging. Energy-dispersive X-ray (EDX) analysis (Fig. S7, ESI†) demonstrated the presence of C, N, O, Na, Sr and Cl. Taken together, these results further confirm that Sr2+ acts as an efficient cation for templating as low as 20 mM 5′-GMP assemblies into high-ordered supramolecular architectures.
Reversible assembly/disassembly mediated by divalent metal ions
Since the G-quartet-based nanowires are harnessed by the presence of a coordinated Sr2+ cation, this offers the possibility of triggering a reversible G-quartet–GMP interconversion. Na2EDTA offers an efficient complexation of Sr2+. The fluorescence spectra for the mixture of 2 μM NMM and 20 mM 5′-GMP were measured in the absence, the presence of 8 mM Sr2+, and the presence of both 8 mM Sr2+ and 10 mM Na2EDTA. As shown in Fig. 3, the fluorescence spectra indicated the assembly and disassembly of nanowires in the presence or absence of Sr2+, respectively. Also no fluorescence signal was shown in the presence of both Sr2+ and Na2EDTA, demonstrating disassembly of the functional wires. It is noteworthy that the spectrum obtained in the presence of both Sr2+ and Na2EDTA is almost identical to that measured in the absence of Sr2+, suggesting that Na2EDTA can efficiently reverse the Sr2+ mediated 5′-GMP supramolecular assembly.
![Fluorescence spectra of NMM mixed with 5′-GMP in deionized water (black) and in the presence of 8 mM SrCl2 (red), or 8 mM SrCl2 + 10 mM Na2EDTA (blue) at ambient temperature; [NMM] = 2 × 10−6 M, [GMP] = 2 × 10−2 M. Inset: Images of the mixtures under UV irradiation with no extra agent (a), 8 mM SrCl2 (b) or 8 mM SrCl2 + 10 mM Na2EDTA (c) at ambient temperature.](/image/article/2011/SC/c1sc00109d/c1sc00109d-f3.gif) |
| Fig. 3
Fluorescence spectra of NMM mixed with 5′-GMP in deionized water (black) and in the presence of 8 mM SrCl2 (red), or 8 mM SrCl2 + 10 mM Na2EDTA (blue) at ambient temperature; [NMM] = 2 × 10−6 M, [GMP] = 2 × 10−2 M. Inset: Images of the mixtures under UV irradiation with no extra agent (a), 8 mM SrCl2 (b) or 8 mM SrCl2 + 10 mM Na2EDTA (c) at ambient temperature. | |
Furthermore, the images of a mixture of 5′-GMP and NMM in aqueous solution under UV irradiation also confirmed this reversible interconversion (Fig. 3, inset). To obtain further evidence for the reversible switch of nanowire assembly SEM measurements were carried out. As can be seen in Fig. 4a, nanowires were not observed in the absence of Sr2+, indicating no high-order structures were assembled. In contrast, regular wires were observed in the presence of Sr2+, which further confirms the finding from fluorescence spectroscopy. Furthermore, no high-order structures were observed in the presence of both Sr2+ and Na2EDTA. Therefore, it was clearly shown that Sr2+ promotes the assembly of 5′-GMP into nanowires and that Na2EDTA reverses the process by chelating with Sr2+. We thus have a novel GMP-based supramolecular architecture that is controllable and can be switched by external signals. Statistical analysis indicated that the length of the wires ranged from several hundreds of nanometres to micrometres and that the average length of the wires (from 100 wires) is 1–1.2 μm (Fig. S3b, ESI†).
![SEM images of functional nanowires of 5′-GMP in deionized water containing: (a) no SrCl2 or Na2EDTA (control), (b) 8 mM SrCl2, (c) 8 mM SrCl2 + 10 mM Na2EDTA; [NMM] = 2 × 10−6 M, [GMP] = 2 × 10−2 M.](/image/article/2011/SC/c1sc00109d/c1sc00109d-f4.gif) |
| Fig. 4
SEM images of functional nanowires of 5′-GMP in deionized water containing: (a) no SrCl2 or Na2EDTA (control), (b) 8 mM SrCl2, (c) 8 mM SrCl2 + 10 mM Na2EDTA; [NMM] = 2 × 10−6 M, [GMP] = 2 × 10−2 M. | |
Application as controlled drug release system
Inspired by this unique reversible feature, we explored the possibility of applying the supramolecular architectures as controlled drug release systems. Two quadruplex-specific ligands were used as model drugs to serve as a proof of concept to test the principle of our design (Scheme 1b). As demonstrated above, addition of 8 mM SrCl2 into 20 mM 5′-GMP solution would promote the G-quartet-based assembly and could simultaneously encapsulate the quadruplex-specific binding molecule NMM, resulting in precipitation from the aqueous solution. Addition of 10 mM Na2EDTA to the system would reverse this process, and as a consequence, the entrapped NMM molecules should be released from the system. This has been verified by fluorescence spectra (Fig. 3); SEM images (Fig. 4) and absorbance spectra of the supernatant (Fig. S8, ESI†).
To further test the utility of our system, another G-quadruplex binder, a cationic perylene tetracarboxylic acid diimide (PTCDI) derivative (Fig. 5c) 42,43 was employed to help visualize the control release process. The PTCDI derivative was introduced into the GMP solution before the assembly process was started. Upon the formation of the high-order supramolecular structure, the PTCDI derivative was trapped within the nanowires and could not be dispersed into the upper layer. Importantly, the resulting PTCDI derivative-containing nanowires are very stable, and no ligand release was observed even after incubation for several days. However, upon the addition of Na2EDTA to chelate the Sr2+, the high-order nanowires disassembled quickly, and the trapped PTCDI derivatives were released and dispersed into the solution within 1 min (Fig. 6). In contrast, neither NMM nor PTCDI derivatives was encapsulated into the precipitates of 5′-IMP/Sr2+, 5′-AMP/Sr2+ and 5′-UMP/Sr2+ systems (Fig. S9 and S10, ESI†). These results were fully consistent with the SEM images for these nucleotides which could not form nanowires under the same conditions (Fig. S11, ESI†).
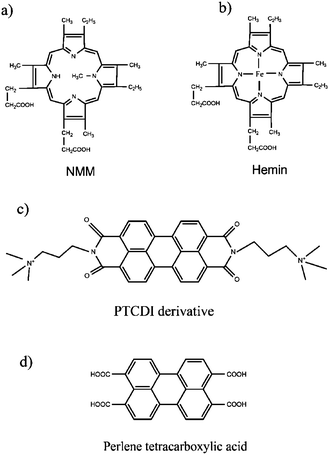 |
| Fig. 5 Chemical structures of (a) NMM, (b) hemin, (c) cationic PTCDI derivative and (d) perylene tetracarboxylic acid. | |
![Encapsulation and release of cationic PTCDI derivative mediated by Sr2+ and Na2EDTA. (A) Absorbance spectra of the supernatant of the mixture of cationic PTCDI derivative and 5′-GMP in deionized water (black) and in the presence of 8 mM SrCl2 (red) or 8 mM SrCl2 + 10 mM Na2EDTA (blue) at ambient temperature. (B) Photographs of cationic PTCDI derivative (a) before and (b) after being trapped in the nanowires and (c) after the addition of 10 mM Na2EDTA leading to release of the PTCDI derivative from the nanowires to form a uniform solution; [PTCDI derivative] = 1 × 10−5 M, [GMP] = 2 × 10−2 M.](/image/article/2011/SC/c1sc00109d/c1sc00109d-f6.gif) |
| Fig. 6 Encapsulation and release of cationic PTCDI derivative mediated by Sr2+ and Na2EDTA. (A) Absorbance spectra of the supernatant of the mixture of cationic PTCDI derivative and 5′-GMP in deionized water (black) and in the presence of 8 mM SrCl2 (red) or 8 mM SrCl2 + 10 mM Na2EDTA (blue) at ambient temperature. (B) Photographs of cationic PTCDI derivative (a) before and (b) after being trapped in the nanowires and (c) after the addition of 10 mM Na2EDTA leading to release of the PTCDI derivative from the nanowires to form a uniform solution; [PTCDI derivative] = 1 × 10−5 M, [GMP] = 2 × 10−2 M. | |
Furthermore, two other small molecules, hemin (Fig. 5b) and perylene tetracarboxylic acid (Fig. 5d), were also tested for the drug encapsulation process. Similar to the cases of NMM and PTCDI derivative, hemin could be also encapsulated into the nanowires since the supernatant showed no absorbance in the Soret band region of porphyrins (Fig. S12a, ESI†). However, for the non/weak-quadruplex binder perylene tetracarboxylic acid which contains four negative –COOH groups, no significant encapsulation was observed (Fig. S12b, ESI†). Meanwhile, the SEM observations showed that the encapsulation of molecules into the assembly has no effect on the morphology of the G-quartet-based nanowires (Fig. S13, ESI†). Therefore, these results indicated that these G-quartet-based nanowires possess the capability for encapsulating only G-quadruplex-specific ligands. To the best of our knowledge, this is a novel controlled quadruplex-specific drug release system by using G-quartet-based supramolecular nanoassembly, which could show potential applications as controlled quadruplex-specific drug release systems.
Conclusions
In conclusion, we have demonstrated a novel strategy to construct metal-mediated G-quartet-based supramolecular architectures with controllable functions. Supramolecular nanowires were prepared by using promoting divalent metal ions and 5′-GMP as basic building blocks in dilute solution. The resulting assemblies have several important properties that make them useful for the development of G-quartet-based functional nanomaterials. First, the Sr2+ promoted assemblies are simple and intuitive, yet cost-effective in eliminating the need of using high concentrations of 5′-GMP and promoting monovalent salts, and/or the need of non-aqueous media. These kind of architectures can be regarded as completely homogeneous from the structural point of view. Second, the nanowires can be controlled by chemical input signals, namely, divalent metal ions and a chelating agent. Third, and importantly, our work demonstrated a new kind of controlled G-quadruplex specific drug delivery and release system by using a G-quartet-based supramolecular nanoassembly. Especially, the encapsulating feature of this system towards G-quadruplex-specific ligands might be of particular biological significance, in the framework of nucleic telomerase. We have previously reported that human telomeric G-quadruplex/i-motif–based assemblies behave as proton-fueled DNA nanosprings that show potential application in drug delivery through covalently incorporated ligand into the system. By using simple molecules such as 5′-GMP and a noncovalent strategy, our current results represent an important step forward in obtaining nucleo-based supramolecular architectures that can be regulated by the surrounding conditions for use as novel functional nanobiomaterials that are switchable, controllable and addressable, and will be highly beneficial for future biochemical, pharmaceutical, drug-delivery, nanomechanical and electronic applications.
Materials and methods
Materials
Hydrated disodium salts of nucleotides: guanosine 5′-monophosphate, Na2(5′-GMP)·xH2O, adenosine 5′-monophosphate, Na2(5′-AMP)·xH2O, uridine 5′-monophosphate, Na2(5′-UMP)·xH2O, inosine 5′-monophosphate, Na2 (5′-IMP)·xH2O and D2O (>99.9 atom%) were purchased from Sigma-Aldrich. The purity of the compounds are 99%. N-Methyl mesoporphyrin IX (NMM) was purchased from Porphyrin Products Inc (Logan, UT), and its concentration was measured by absorbance at 379 nm assuming an extinction coefficient of 1.45 × 105 M−1 cm−1. Other chemicals were purchased from Sigma–Aldrich and used without further purification. All samples were prepared in Milli-Q water, except those for NMR studies. No buffers or other ionic agents were added in order to avoid the introduction of additional assembly-promoting cations to the solution.
Measurements
UV-vis absorption and fluorescence spectra were recorded on a JASCO V-550 spectrophotometer and JASCO FP-6500 spectrofluorometer, respectively. These apparatus are equipped with Peltier type thermostatic cell holders. Quartz cells with 1-cm path lengths were used. Fluorescence microscopy was conducted on an Olympus BX-51 optical system microscope (Tokyo, Japan) at 1000× magnification with a blue filter. Pictures were taken with an Olympus digital camera. Scanning electron microscopy (SEM) was conducted on an S-4800 Field Emission Scanning Microscope (acceleration voltage, 10 kV). Transmission electron microscopy (TEM) was conducted on a JEOL JEM-1011 (acceleration voltage, 100 kV).
Preparation of TEM and SEM samples
A drop of aqueous mixtures of nucleotides (AMP, UMP, IMP and GMP) and SrCl2 is placed on carbon-coated Cu grids. These specimens were dried at ambient temperature and observed by electron microscopy. The preparation of SEM specimens follows the same procedure as TEM but uses silicon slices as substitutes for carbon-coated Cu grids.
Reversible assembly/disassembly process
To three 1.5 ml Eppendorf cups (a, b, c) were added a 400 μl mixture of 20 mM GMP and 2 μM NMM. 8 mM SrCl2 was added to cups (b) and (c), and shaken slightly to form a homogeneous solution. After 24 h incubation at ambient temperature, white fibrous structures were observed in cups (b) and (c). Upon addition of 10 mM Na2EDTA to cup (c) to chelate the Sr2+, the fibrous structure disappeared within one minute. Fluorescence spectra, UV spectra, UV irradiation image and SEM images were observed for the three samples. If 8 mM SrCl2 and 10 mM Na2EDTA were added simultaneously or in reversed order the assembly process was also inhibited and no fibrous structure could be observed.
Acknowledgements
This work was supported by the National Basic Research Program of China (Grant 2011CB936004) and the National Natural Science Foundation of China (Grants 20831003, 90813001, 20833006, 90913007 and 21072182).
Notes and references
- A. T. Bell, Science, 2003, 299, 1688–1691 CrossRef CAS.
- M. H. Wu and G. M. Whitesides, Appl. Phys. Lett., 2001, 78, 2273–2275 CrossRef CAS.
- M. A. Morikawa, M. Yoshihara, T. Endo and N. Kimizuka, J. Am. Chem. Soc., 2005, 127, 1358–1359 CrossRef CAS.
- T. Shiraki, M. A. Morikawa and N. Kimizuka, Angew. Chem., Int. Ed., 2008, 47, 106–108 CrossRef CAS.
- R. Otero, W. Xu, M. Lukas, R. E. Kelly, E. Laegsgaard, I. Stensgaard, J. Kjems, L. N. Kantorovich and F. Besenbacher, Angew. Chem., Int. Ed., 2008, 47, 9673–9676 CrossRef CAS.
- M. Gellert, M. N. Lipsett and D. R. Davies, Proc. Natl. Acad. Sci. U. S. A., 1962, 48, 2013–2018 CAS.
- J. T. Davis, Angew. Chem., Int. Ed., 2004, 43, 668–698 CrossRef CAS.
- J. T. Davis and G. P. Spada, Chem. Soc. Rev., 2007, 36, 296–313 RSC.
- S. Lena, S. Masiero, S. Pieraccini and G. P. Spada, Chem.–Eur. J., 2009, 15, 7792–7806 CrossRef CAS.
- M. S. Kaucher, W. A. Harrell, Jr. and J. T. Davis, J. Am. Chem. Soc., 2006, 128, 38–39 CrossRef CAS.
- C. Arnal-Herault, A. Pasc, M. Michau, D. Cot, E. Petit and M. Barboiu, Angew. Chem., Int. Ed., 2007, 46, 8409–8413 CrossRef CAS.
- C. Arnal-Herault, A. Banu, M. Barboiu, M. Michau and A. van der Lee, Angew. Chem., Int. Ed., 2007, 46, 4268–4272 CrossRef CAS.
- A. Ghoussoub and J. M. Lehn, Chem. Commun., 2005, 5763–5765 RSC.
- N. Sreenivasachary and J. M. Lehn, Chem.–Asian J., 2008, 3, 134–139 CrossRef CAS.
- J. E. Betancourt and J. M. Rivera, J. Am. Chem. Soc., 2009, 131, 16666–16668 CrossRef CAS.
- J. E. Betancourt, C. Subramani, J. L. Serrano-Velez, E. Rosa-Molinar, V. M. Rotello and J. M. Rivera, Chem. Commun., 2010, 46, 8537–8539 RSC.
- S. Pieraccini, S. Masiero, O. Pandoli, P. Samori and G. P. Spada, Org. Lett., 2006, 8, 3125–3128 CrossRef CAS.
- S. Pieraccini, S. Bonacchi, S. Lena, S. Masiero, M. Montalti, N. Zaccheroni and G. P. Spada, Org. Biomol. Chem., 2010, 8, 774–781 RSC.
- A. Ciesielski, S. Lena, S. Masiero, G. P. Spada and P. Samori, Angew. Chem., Int. Ed., 2010, 49, 1963–1966 CAS.
- S. Lena, P. Neviani, S. Masiero, S. Pieraccini and G. P. Spada, Angew. Chem., Int. Ed., 2010, 49, 3657–3660 CAS.
- E. Bouhoutsos-Brown, C. Marshall and T. J. Pinnavaia, J. Am. Chem. Soc., 1982, 104, 6576–6584 CrossRef CAS.
- A. Wong, R. Ida, L. Spindler and G. Wu, J. Am. Chem. Soc., 2005, 127, 6990–6998 CrossRef CAS.
- G. Wu and I. C. Kwan, J. Am. Chem. Soc., 2009, 131, 3180–3182 CrossRef CAS.
- R. Ida, I. C. Kwan and G. Wu, Chem. Commun., 2007, 795–797 RSC.
- A. Wong and G. Wu, J. Am. Chem. Soc., 2003, 125, 13895–13905 CrossRef CAS.
- J. B. Hightower, D. R. Olmos and J. A. Walmsley, J. Phys. Chem. B, 2009, 113, 12214–12219 CrossRef CAS.
- F. M. Chen, Biochemistry, 1992, 31, 3769–3776 CrossRef CAS.
- T. C. Marsh, J. Vesenka and E. Henderson, Nucleic Acids Res., 1995, 23, 696–700 CrossRef CAS.
- D. Miyoshi, A. Nakao and N. Sugimoto, Nucleic Acids Res., 2003, 31, 1156–1163 CrossRef CAS.
- B. I. Kankia and L. A. Marky, J. Am. Chem. Soc., 2001, 123, 10799–10804 CrossRef CAS.
- I. M. Pedroso, L. F. Duarte, G. Yanez, A. M. Baker and T. M. Fletcher, Biochem. Biophys. Res. Commun., 2007, 358, 298–303 CrossRef CAS.
- G. Wu and A. Wong, Biochem. Biophys. Res. Commun., 2004, 323, 1139–1144 CrossRef CAS.
- A. Wong, R. Ida and G. Wu, Biochem. Biophys. Res. Commun., 2005, 337, 363–366 CrossRef CAS.
- J. Ren and J. B. Chaires, Biochemistry, 1999, 38, 16067–16075 CrossRef CAS.
- H. Arthanari, S. Basu, T. L. Kawano and P. H. Bolton, Nucleic Acids Res., 1998, 26, 3724–3728 CrossRef CAS.
- D. Hu, F. Pu, Z. Huang, J. Ren and X. Qu, Chem.–Eur. J., 2010, 16, 2605–2610 CrossRef CAS.
- D. Hu, Z. Huang, F. Pu, X. Qu and J. Ren, Chem.–Eur. J., 2011, 17, 1635–1641 CrossRef CAS.
- J. Deng, Y. Xiong and M. Sundaralingam, Proc. Natl. Acad. Sci. U. S. A., 2001, 98, 13665–13670 CrossRef CAS.
- X. Shi, J. C. Fettinger and J. T. Davis, Angew. Chem., Int. Ed., 2001, 40, 2827–2831 CrossRef CAS.
- E. R. Henderson, M. Moore and B. A. Malcolm, Biochemistry, 1990, 29, 732–737 CrossRef CAS.
- C. Detellier and P. Laszlo, J. Am. Chem. Soc., 1980, 102, 1135–1141 CrossRef CAS.
- H. Han, C. L. Cliff and L. H. Hurley, Biochemistry, 1999, 38, 6981–6986 CrossRef CAS.
- J. T. Kern, P. W. Thomas and S. M. Kerwin, Biochemistry, 2002, 41, 11379–11389 CrossRef CAS.
Footnote |
† Electronic supplementary information (ESI) available: Fig. S1–S13, Table S1. See DOI: 10.1039/c1sc00109d |
|
This journal is © The Royal Society of Chemistry 2011 |
Click here to see how this site uses Cookies. View our privacy policy here.