DOI:
10.1039/C1AN15457E
(Paper)
Analyst, 2012,
137, 195-201
Investigation of the toxic effect of a QDs heterojunction on the interactions between small molecules and plasma proteins by fluorescence and resonance light-scattering spectra†
Received
3rd June 2011
, Accepted 22nd September 2011
First published on 2nd November 2011
Abstract
The effect of a ZnO#ZnS QDs heterojunction (O#SQDs) on the binding affinities of flavonoid glycosides for bovine serum albumin (BSA) was investigated. The fluorescence intensities of BSA decreased remarkably with increasing concentration of O#SQDs. The magnitudes of the binding constants of flavonoid glycosides for BSA in the presence of O#SQDs were in the range of 105–107 L mol−1, and the number of binding sites per BSA (n) was determined as 1.24 ± 0.17. O#SQDs increased the affinities of flavonoid glycosides for BSA by about 2.96% to 114.68% depending on their structures. O#SQDs in blood will enhance the transportation of flavonoid glycosidegs in blood and improve their pharmacology effects. From this point, O#SQDs are a perfect candidate for flavonoid glycosides delivery applications.
1. Introduction
Dietary flavonoids are very important polyphenols in plant foods such as fruits, vegetables, nuts, and tea.1–5 Investigation of flavonoids from dietary sources has attracted great interest for their nutritional and medical effects on humans. The structural difference of flavones significantly affects their absorption, metabolism, and bioactivities.6–10 The dietary flavonoids in nature exist almost as β-glycosides.11 The flavonols are found mainly as the 3-O-glycoside, although the 7 and 4′ positions may also be glycosylated in some plants.12 Other classes of favonoids, such as favone, favanone and isofavone, are found mainly glycosylated in the 7 position.11 The dietary flavonoid glycosides in most cases are hydrolyzed to aglycones to produce effects.13
Colloidal nanocrystals (NCs) or quantum dots (QDs) are colloidal semiconductor nanoparticles with unique luminescence characteristics and wide biological applications.14,15CdTe, CdS/ZnSe, CdSe/CdTe and CdS/CdSe QDs are widely studied due to their unique optical and electronic properties such as wide and continuous absorption spectra, narrow emission spectra, and high light stability. However, these Cd-based QDs showed obvious biological toxicity and and their biological applications are doubtful.16,17 Therefore, a less toxic or nontoxic QDs system is necessary to replace the Cd-based QDs. Zero-dimensional nanostructures such as in a ZnO#ZnS QDs heterojunction (O#SQDs) are green nanoparticles and have gained a tremendous amount of attention in recent years due to their fascinating chemistry and size-, shape-, and material-dependent properties.
The serum albumins are the major transport proteins in blood. The most outstanding property of serum albumins is the ability to bind small molecules reversibly, such as fatty acids, amino acids, drugs, and inorganic ions.18 The interaction between drugs and serum albumins has important effects on the distribution, free concentration and metabolism of drugs in the blood stream.19 Recently, investigations of the interaction of CdTe QDs with serum albumins have attracted great interest for their drug nanocarriers applications.20–22 However, very little information is available on whether or not O#SQDs affect the transportation of drugs in blood. And few reports have focused on the toxic effect of a QDs heterojunction on the interaction between small molecules and plasma proteins. Fluorescence quenching spectroscopy is an appropriate method to determine the binding parameters between drugs and proteins. Herein, six flavonoid glycosides (Fig. 1) were studied for their affinities for bovine serum albumin (BSA) in the presence and absence of O#SQDs.
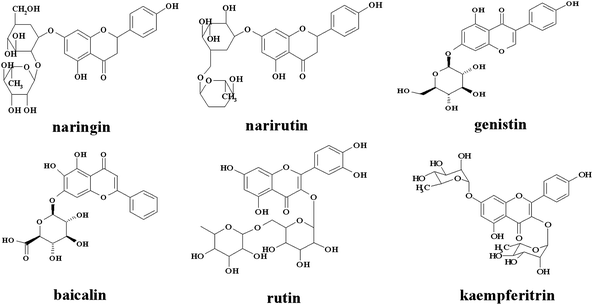 |
| Fig. 1 Structures of flavonoid glycosides. | |
2. Experimental
2.1 Reagents
Genistin, baicalin, naringin, rutin, kaempferitrin and narirutin (>98.0%) were obtained commercially from Shanghai Tauto Biotech CO., Ltd (Shanghai, China) and used without further purification. The working solutions of flavonoids (1.0 × 10−3 mol L−1) were prepared by dissolving flavones with methanol. BSA (fraction V) was purchased from Sigma Co. (MO, USA). The working solution of BSA (1.0 × 10−5 mol L−1) was prepared with PBS buffer and stored in a refrigerator prior to use. Zinc acetate dehydrate (ZnAc2, AR), thiacetamide (AR), tetraethoxysilane (TEOS, AR), ammonia (AR) and 1,4-butanediol (1,4-BD, AR) were purchased from Aladdin agents Co. (Shanghai, China). All other reagents and solvents were of analytical reagent grade and used without further purification unless otherwise noted. All aqueous solutions were prepared using newly double-distilled water.
2.2. Apparatus
Fluorescence experiments were performed on a Varian Cary/E spectrofluorophotometer (Palo Alto, USA). UV-vis absorption spectra were carried out on a Varian Cary 50 UV-vis spectrometer (Palo Alto, USA). The transmission electron microscopy (TEM) measurement was carried out on a Hitachi H-600 TEM (Tokyo, Japan). Magnetic stirring was carried out using an IKA RH KT/C magnetic stirrer (Staufen, Germany).
2.3. Preparation of O#SQDs
The preparation of O#SQDs was according to our previous report.23 In a typical experiment, 2 mmol of ZnAc and 1.4 mmol of CH3CSNH2 were added to 20 mL of 1,4-BD and dispersed by ultrasonic power until a transparent solution was formed. The formed solution was transferred into a 30 mL Teflon-lined stainless steel autoclave. Solvothermal treatments were carried out at 200 °C for 18 h. After the autoclave was allowed to cool down to room temperature naturally, the products were precipitated by adding distilled water and separated by centrifugation at the speed of 8000 rpm. The collected white colloids were washed with ethanol three times to remove impurities. Finally, the precipitates were dried in air at 60 °C for 5 h to obtain O#SQDs. And then the O#SQDs were coated with silica. O#SQDs (0.1 mmol) were dispersed in ethanol (40 mL) and mixed with ammonia (28%, 0.5 mL). A mixture of TEOS (0.1 mL) and ethanol (10 mL) was then added slowly to the solution with continuous stirring. The reaction time is about 12 h. The product was isolated by centrifugation and washed twice with water.
A 3.0 mL working solution of BSA (1.0 × 10−5 mol L−1) was transferred to a 1.0 cm quartz cell. And then it was titrated with successive addition of 3.0 μl polyphenol solution (1.0 × 10−3 mol L−1) or O#SQDs. Titrations were performed manually by using trace syringes at 37 °C. In each titration, the fluorescence spectrum was collected with the working solution of BSA (1.0 × 10−5 mol L−1). The fluorescence spectra were recorded in the wavelength range of 310–450 nm upon excitation at 280 nm when BSA (1.0 × 10−5 mol L−1) was titrated with polyphenols solution or O#SQDs. Slit widths, scan speed and excitation voltage were kept constant within each data set and each spectrum was the average of three scans. The results of the time course experiments for the equilibration are not given here. The fluorescence emissions of these polyphenols within the range of 300–400 nm were not observed under the excitation wavelength of 280 nm. The polyphenols were stable during the fluorescence measurements, as shown by HPLC analyses (not given here). Each fluorescence intensity determination was repeated three times and found to be reproducible within experimental errors (<5%).
2.5. Determination of binding parameters
Fluorescence quenching was described by the Stern–Volmer equation:24–27 | F0/F = 1 + Kqτ0 [Q] =1 + KSV [Q] | (1) |
where F0 and F represent the fluorescence intensities of the fluorophore in the absence and in the presence of the quencher, Kq is the quenching rate constant of the bimolecular, KSV is the dynamic quenching constant, τ0 is the average lifetime of the fluorophore without quencher, and [Q] is the concentration of the quencher.
In many instances, the fluorophore can be quenched both by collision and by complex formation with the same quencher. In this case, the Stern–Volmer plot exhibits an upward curvature, concave towards the y-axis at high [Q], and F0/F is related to [Q] by the modified form of the Stern–Volmer equation:24,25
| F0/F=(1 + KD[Q]) (1 + KS[Q]) | (2) |
where
KD and
KS are the dynamic and static quenching constants, respectively.
The binding constants were calculated according to the double-logarithm equation:24–27
| lg[(F0 − F)/F]= lgKa + n lg[Q] | (3) |
where
Ka is the binding constant and
n is the number of binding sites per BSA. From
fluorescence titration, the values of “(
F0 −
F)/
F” can be obtained in each “[
Q]”. And then, the linear regression equation between the “lg[(
F0 −
F)/
F]” values and “lg[
Q]” values was obtained on the Origin 7.5 software. The slope factor means “
n” and the intercept refers to “lg
Ka”.
2.6. Resonance light-scattering spectra
An appropriate aliquot of BSA working solution was added to 3.0 ml 1.67 × 10−5 mol L−1 of O#SQDs solution. RLS spectra were obtained by synchronous scanning in the wavelength range of 300–750 nm on the spectrofluorophotometer. The spectral bandwidths of the excitation and emission monochromators were both kept at 3.0 nm. The operations were carried out at room temperature.
3. Results and discussion
3.1 Characterization of O#SQDs
The O#SQDs overcoated by SiO2 were analyzed by XRD (Supplementary material 1).† All the diffraction peaks in the XRD patterns can be attributed to hexagonal wurtzite-8H ZnS (JCPDS Card No. 39-1363) and hexagonal wurtzite ZnO QDs (JCPDS Card No. 36-1451). They have the chance to grow together into a ZnS/ZnO heterostructure. In order to obtain more detailed information about the micro-structure and morphology of the synthesized samples, TEM observations were carried out. Supplementary material 2 shows the TEM picture of O#SQDs.† The amplified O#SQDs in Supplementary material 2 show the high-resolution TEM (HRTEM) image of O#SQDs marked by a black circle. The growth direction of the O#SQDs was observed. The lattice fringes of the wurtzite ZnS (0008) planes with d-spacings of ≈0.31 nm can be clearly seen in the low portion of the QDs, and the lattice fringes of the wurtzite ZnO (0002) planes with the d-spacing of ≈0.26 nm of the wurtzite ZnO were observed in the top portion. The heterojunction shows the growth direction in the QDs range. Normally, the O#SQDs tend to aggregate together under this critical solvent thermal synthesizing condition. However, the ZnS and ZnO QDs are still dispersed separatively in our case. Supplementary material 2 shows the diameters of O#SQDs were about 10 nm and the particle size distribution of O#SQDs was uniform.†
3.2 O#SQDs–BSA Interaction
BSA has three intrinsic fluorophores: tryptophan, tyrosine and phenylalanine. Because the quantum yield of phenylalanine is very low and the fluorescence of tyrosine is almost totally quenched, the intrinsic fluorescence of BSA is almost entirely due to tryptophan. When the fluorescence emission spectra of BSA are measured with a series of concentrations of quencher by fixing the excitation wavelength at 280 nm, the fluorescence emission peak of BSA at 340 nm mainly gives the information of Trp residues.
The fluorescence spectra of BSA with addition of O#SQDs are shown in Fig. 2. The fluorescence intensities of BSA decreased remarkably with increasing concentration of O#SQDs. In addition, the O#SQDs resulted in an obvious red-shift of the maximum emission of BSA from 340 to 348 nm. These results suggested that there was a change in the immediate environment of the tryptophan residues and that the O#SQDs were situated at close proximity to the tryptophan residues for the quenching to occur. In the present study, the information about other amino acid residues was not understood. The buried indole groups could be re-deployed in a more hydrophobic environment after addition of O#SQDs.
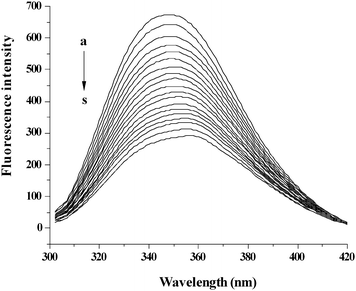 |
| Fig. 2 The quenching effect of O#SQDs on BSA fluorescence intensity. λex = 280 nm; BSA, 1.00 × 10−5 mol L−1; a–s: 0.00, 2.00, 4.00… 36.00 (× 10−6 mol L−1) of O#SQDs. | |
When the concentration of O#SQDs approached to 30 μmol L−1, the fluorescence intensity of BSA hardly decreased. Approximately 51.04% of the BSA fluorescence (F/F0) was quenched when 30 μmol L−1 of O#SQDs was added (Supplementary material 3).† For further discussion, the concentration of O#SQDs was fixed as 15 μM. The Stern–Volmer plots (Supplementary material 4)† significantly deviated from linearity toward the y-axis at high O#SQDs concentration, which indicated that both dynamic and static quenching were involved for O#SQDs on BSA fluorescence. The binding constant and the number of binding sites between O#SQDs and BSA were determined as 7.41 × 104 L mol−1 and 1.08 according to the double-logarithm curves (eqn (3)).
3.3 Characteristics of the RLS spectra
The RLS spectra of O#SQDs solution (1.67 × 10−5 mol L−1) are shown in Fig. 3. Upon addition of a trace amount of BSA to the O#SQDs solution, a remarkably increased RLS with a maximum peak appeared at 467 nm. The production of RLS is correlated with the formation of an aggregate and the RLS intensity is dominated primarily by the particle dimension of the formed aggregate. Bearing these points in mind, it is inferred from the results that the added proteins may interact with O#SQDs in solution, forming a new stable O#SQDs-protein complex that could be expected to be an aggregate. The newly formed O#SQDs-protein complex may be ascribed to the higher electrostatic attraction. The dimension of the resultant O#SQDs-protein particles may be bigger than that of O#SQDs, and thus the increased light-scattering signal occurred under the given conditions.
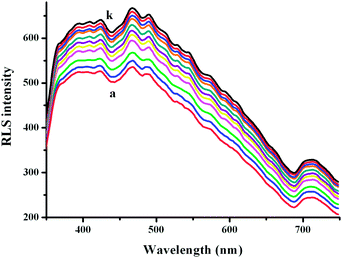 |
| Fig. 3 RLS spectra of 3 ml 1.67 × 10−5 M of O#SQDs with 0 (a), 10 (b),… 100 μl (k) BSA (1.00 × 10−5 mol L−1). | |
3.4 Interaction of BSA with flavonoid glycosides
The weak interaction of BSA with flavonoid glycosides has been studied widely by our group .24–27 In general, the glycosylation of flavonoids lowers the affinity for BSA by 1 to 3 orders of magnitude depending on the conjugation site and the class of sugar moiety.24 In previous reports, appropriate quantities of flavonoid glycosides solution were mixed with BSA and subsequently incubated at 300.15 K for 1 h. Then, the solution was scanned on a fluorometer. However, in the current work, the fluorescence titration method was carried out. The fluorescence spectrum was collected with the concentrations of BSA at 1.0 × 10−5 mol L−1. The binding affinities obtained by these two methods are slightly different.
Upon titration of flavonoid glycosides to the BSA solution, the fluorescence quenching of BSA occurred. The fluorescence intensity attenuated gradually with increasing concentration of flavonoid glycosides. Approximately 32.68%, 13.17%, 21.80%, 14.54%, 27.11% and 32.08% of BSA fluorescence quenching were observed when the concentration of baicalin, genistin, naringin narirutin, kaempferitrin and rutin reached to 8.0 μM (Fig. 4). The binding constants and the number of binding sites between flavonoid glycosides and BSA according to the double-logarithm equation are shown in Table 1.
Table 1 The binding constants (lgKa) for flavonoid-BSA in the absence and presence of O#SQDs
Flavonoid
glycosides
|
Absence |
Presence |
K
a (L mol−1) |
n
|
R
2
|
K
a (L mol−1) |
n
|
R
2
|
baicalin
|
7.41(±1.21) × 104 |
1.02 |
0.9979 |
2.82(±1.14) × 106 |
1.28 |
0.9996 |
genistin
|
1.86(±0.87) × 103 |
0.81 |
0.9991 |
1.05(±0.72) × 107 |
1.41 |
0.9954 |
kaempferitrin
|
1.23(±0.76) × 105 |
1.08 |
0.9973 |
5.25(±3.24) × 107 |
1.14 |
0.9993 |
naringin
|
3.55(±1.13) × 103 |
0.81 |
0.9992 |
1.82(±1.46) × 105 |
1.10 |
0.9989 |
narirutin
|
1.66(±1.38) × 103 |
0.78 |
0.9978 |
9.12(±4.39) × 104 |
1.08 |
0.9981 |
rutin
|
1.15(±0.99) × 105 |
1.06 |
0.9982 |
1.62(±0.68) × 105 |
1.06 |
0.9989 |
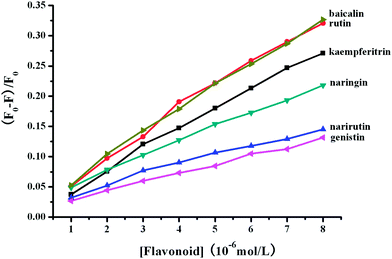 |
| Fig. 4 The quenching effects ((F0 − F)/F0) of BSA fluorescence with addition of flavonoid glycosides. | |
3.5 Fluorescence quenching of BSA induced by flavonoid glycosides in the presence of O#SQDs
When flavonoid glycosides were continuously added to the BSA solution (1.00 × 10−5 M) containing 30 μmol L−1 of O#SQDs, further attenuation in the fluorescence of BSA was observed (Supplementary material 5).† In addition, the obvious red-shifts of the maximum emission of BSA with the addition of flavonoid glycosides were also observed in the presence of O#SQDs. However, the extents of shifts induced by flavonoid glycosides in the presence of O#SQDs were much smaller than those in the absence of O#SQDs. The shifts of maximum λem of BSA induced by baicalin, genistin, naringin, narirutin and rutin were 2, 3, 2, 1 and 0 nm in the presence of O#SQDs, respectively. The quenching effects ((F0 − F)/F0) of BSA fluorescence with addition of flavonoid glycosides in the presence of O#SQDs are shown in Fig. 5. As seen from these data, approximately 46.58%, 43.88%, 43.23%, 39.24%, 32.29% and 21.24% of BSA fluorescence quenching were observed when the concentration of baicalin, genistin, kaempferitrin, rutin, naringin and narirutin reached to 8.0 μM. Comparing Fig. 5 with Fig. 4, O#SQDs significantly enhanced the quenching effects of BSA fluorescence induced by these flavonoid glycosides.
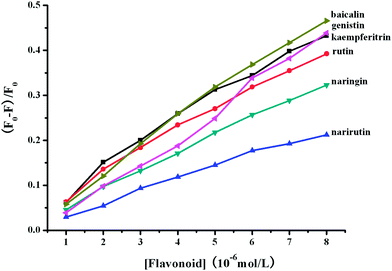 |
| Fig. 5 The quenching effects ((F0 − F)/F0) of BSA-O#SQDs fluorescence with addition of flavonoid glycosides. | |
Fig. 6 shows the Stern–Volmer plots for the BSA fluorescence quenching by flavonoid glycosides in the presence of O#SQDs. As shown in Fig. 6, the Stern–Volmer plots largely deviated from linearity toward the y-axis at high concentrations of baicalin, genistin, and naringin. The Stern–Volmer plots for narirutin and rutin are linear. These results indicated that the flavonoid structure affected the binding interaction between flavonoid and proteins.
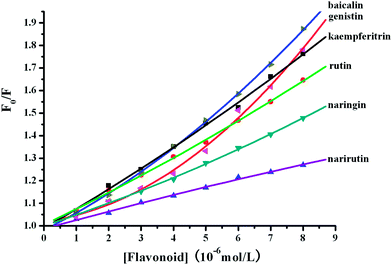 |
| Fig. 6 The Stern–Volmer plots for BSA fluorescence quenching by flavonoid glycosides in the presence of O#SQDs. | |
3.6 Effect of ZnO#ZnS QDs heterojunction on the affinities of flavonoid glycosides for BSA
Fig. 7 shows the double-logarithm curves of flavonoid glycosides quenching BSA fluorescence in the presence of O#SQDs and Table 1 gives the corresponding calculated results. The magnitudes of binding constants in the presence of O#SQDs were in the range of 105–107 L mol−1, which were significantly higher than the common affinities of drugs for serum albumins (104–106 L mol−1) in vivo .28 The number of binding sites per BSA (n) was determined as 1.24 ± 0.17, which indicated that one binding site formed between the flavonoid glycosides and BSA. The relationship between the lgKa and the number of binding sites (n) between flavonoid glycosides and BSA in the absence and presence of O#SQDs is shown in Fig. 8. The values of lgKa are proportional to the number of binding sites (n). This result confirms the eqn (3) used here is suitable to study the interaction between flavonoid glycosides and BSA. As shown in Fig. 9, O#SQDs increased the affinities of flavonoid glycosides for BSA by 2.96% to 114.68% depending on their structures. The affinities of genistin, naringin and narirutin for BSA were significantly improved. However, the affinity of rutin for BSA was hardly changed.
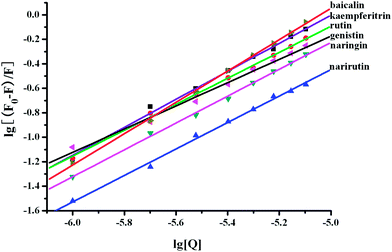 |
| Fig. 7 Double-logarithm curves of flavonoid glycosides quenching BSA fluorescence in the presence of O#SQDs. | |
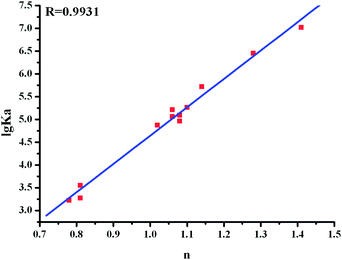 |
| Fig. 8 Relationship between lgKa and the numbers of binding sites (n) between flavonoid glycosides and BSA. | |
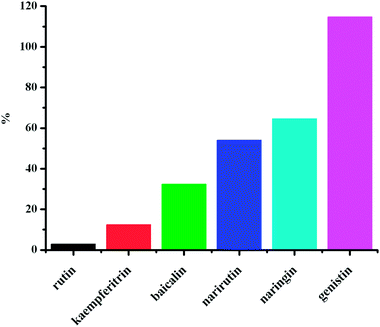 |
| Fig. 9 O#SQDs enhanced the affinities of flavonoid glycosides for BSA. | |
The lower affinities of flavonoid glycosides for BSA in the absence of O#SQDs may be caused by the increasing molecular size and polarity, decreasing the hydrophobicity and transfering to the non-planar structure.24 After the hydroxyl group is substituted by a glycoside, steric hindrance may take place, which weakens the affinity for BSA.24 In many proteins, the binding of the first ligand to the protein can change the affinity for the second ligand, which is called the cooperative effect. The cooperative effect can be divided into the positive and negative cooperative effect. Positive cooperativity is a phenomenon in which binding at one site interferes with binding to additional, identical sites on the protein. It was hypothesized that O#SQDs may show positive cooperativity for flavonoid glycosides when bound to BSA together. The reasons for positive cooperativity may be due to the conformational changes induced by ligand binding and the favorable interactions between ligands. The further investigation and discussion will be provided in the future.
Plants usually glycosylate metabolites to enhance their solubility and improve sequestration into specific cellular compartments. Deglycosylation normally generates the active form of the compound. Many researchers have proved that flavonoid aglycones have stronger bioactivity than their glycosidesin vivo.29,30 The lower affinities for plasma protein may be one of the explanations for the decreased pharmacological effects of flavonoid glycosides. According to the free drug hypothesis, the distribution of a drug within the body is generally held to be driven by the free concentration of unbound drug in circulating plasma.31 If a molecule is weakly bound to plasma proteins, the transportation of the drug in the blood may be significantly reduced and the efficacy of the drug may consequently be poor. Here, it was found that O#SQDs increased the affinities of flavonoid glycosides for serum albumin. O#SQDs in blood will affect the transporting ability of serum albumin for flavonoid glycosides, which may enhance the pharmacological effects of flavonoid glycosides. From this point, O#SQDs are a perfect candidate for flavonoid glycosides delivery applications.
4. Conclusion
Recently, investigations of the interaction of QDs with serum albumins have attracted great interest for their drug nanocarriers applications. CdTe QDs have numerous potential technological applications but may be hazardous as a result of a variety of interactions with biological systems possibly leading to harmful effects.32–34 Zero-dimensional nanostructures such as ZnO#ZnS QDs heterojunctions have gained a tremendous amount of attention in recent years. In the present work, the flavonoid glycosides were studied for their affinities for BSA in the presence and absence of O#SQDs in vitro. A ZnO#ZnS QDs heterojunction showed positive cooperativity for flavonoid glycosides when bound to BSA together. O#SQDs increased the affinities of flavonoid glycosides for BSA by about 2.96% to 114.68% depending on their structures. It was hypothesized that O#SQDs in blood will enhance the transportation of flavonoid glycoside drugs in the blood and improve their pharmacological effects. From this point, O#SQDs are a perfect candidate for flavonoid glycosides delivery applications.
Acknowledgements
The authors are grateful for financial sponsoring by the Shanghai Rising-Star Program (11QA1404700), Shanghai Science and Technology Development Fund (no. 09520500500), the Key Laboratory of Resource Chemistry of Ministry of Education of China, and the Scientific Innovation Fund of the Shanghai Education Commission (09ZZ136 and 10YZ68), Natural Science Foundation of Shanghai (10ZR1421700), Leading Academic Discipline Project of Shanghai Municipal Education Commission (J50401), “Chen Guang” project supported by Shanghai Municipal Education Commission and Shanghai Education Development Foundation (09CG46), and Program of Shanghai Normal University (SK201006).
References
- S. D. Cohen and J. A. Kennedy, Plant metabolism and the environment: Implications for managing phenolics, Crit. Rev. Food Sci. Nutr., 2010, 50, 620–543 CrossRef CAS.
- J. Côté, S. Caillet, G. Doyon, J. F. Sylvain and M. Lacroix, Analyzing cranberry bioactive compounds, Crit. Rev. Food Sci. Nutr., 2010, 50, 872–888 CrossRef.
- C. W. Y. Cheung, N. Gibbons, D. W. Johnson and D. L. Nicol, Silibinin - A promising new treatment for cancer, Anti-cancer Agents Med Chem., 2010, 10, 186–195 CAS.
- J. Côté, S. Caillet, G. Doyon, J. F. Sylvain and M. Lacroix, Bioactive compounds in cranberries and their biological properties, Crit. Rev. Food Sci. Nutr., 2010, 50, 666–679 CrossRef.
- S. Arranz, J. M. Silván and F. Saura-Calixto, Nonextractable polyphenols, usually ignored, are the major part of dietary polyphenols: A study on the Spanish diet, Mol. Nutr. Food Res., 2010, 54, 1646–1658 CAS.
- W. Brand, B. Padilla, P. J. van Bladeren, G. Williamson and I. M. C. M. Rietjens, The effect of co-administered flavonoids on the metabolism of hesperetin and the disposition of its metabolites in Caco-2 cell monolayers, Mol. Nutr. Food Res., 2010, 54, 851–860 CAS.
- C. Blade, L. Arola and M. Salvado, Hypolipidemic effects of proanthocyanidins and their underlying biochemical and molecular mechanisms, Mol. Nutr. Food Res., 2010, 54, 37–59 CAS.
- G. Morabito, D. Trombetta, K. S. Brajendra, K. P. Ashok, S. P. Virinder and C. Naccari,
et al., Antioxidant properties of 4-methylcoumarins in in vitro cell-free systems, Biochimie, 2010, 92, 1101–1107 CrossRef CAS.
- F. Natella, B. Lorrain, A. K. Prasad, V. S. Parmar, L. Saso and C. Scaccini, 4-Methylcoumarins as antioxidants: Scavenging of peroxyl radicals and inhibition of human low-density lipoprotein oxidation, Biochimie, 2010, 92, 1147–1152 CrossRef CAS.
- G. Basini, C. Tringali, L. Baioni, S. Bussolati, C. Spatafora and F. Grasselli, Biological effects on granulosa cells of hydroxylated and methylated resveratrol analogues, Mol. Nutr. Food Res., 2010, 54, S236–S243 CAS.
- A. J. Day, M. S. DuPont, S. Ridley, M. Rhodes, M. J. C. Rhodes and M. R. A. Morgan,
et al., Deglycosylation of favonoid and isofavonoid glycosides by human small intestine and liver L-glucosidase activity, FEBS Lett., 1998, 436, 71–75 CrossRef CAS.
- T. Fossen, A. T. Pedersen and M. Anderson, Flavonoids from red onion (Allium cepa), Phytochemistry, 1998, 47, 281–285 CrossRef CAS.
- T. Walle, A. M. Browning, L. L. Steed, S. G. Reed and U. K. Walle, Flavonoid glucosides are hydrolyzed and thus activated in the oral cavity in humans, J Nutr, 2005, 135, 48–52 CAS.
- B. S. Sekhon and S. R. Kamboj, Inorganic nanomedicine-Part 2, Nanomed.: Nanotechnol., Biol. Med., 2010, 6, 612–618 CrossRef CAS.
- B. S. Sekhon and S. R. Kamboj, Inorganic nanomedicine-Part 1, Nanomed.: Nanotechnol., Biol. Med., 2010, 6, 516–522 CrossRef CAS.
- T. Skotland, T. G. Iversen and K. Sandvig, New metal-based nanoparticles for intravenous use: requirements for clinical success with focus on medical imaging, Nanomed.: Nanotechnol., Biol. Med., 2010, 6, 730–737 CrossRef CAS.
- B. Mishra, B. B. Patel and S. Tiwari, Colloidal nanocarriers: a review on formulation technology, types and applications toward targeted drug delivery, Nanomed.: Nanotechnol., Biol. Med., 2010, 6, 9–24 CrossRef CAS.
- J. Xiao, X. Chen, L. Zhang, S. G. Talbot, G. C. Li and M. Xu, Investigation the mechanism of enhanced effect of EGCG on huperzine A inhibiting acetylcholinesterase activity in rats by multi-spectroscopic method, J. Agric. Food Chem., 2008, 56, 910–915 CrossRef CAS.
- S. H. Cao, X. Y. Jiang and J. W. Chen, Effect of Zinc (II) on the interactions of bovine serum albumin with flavonols bearing different number of hydroxyl substituent on B-ring, J. Inorg. Biochem., 2010, 104, 146–152 CrossRef CAS.
- J. B. Xiao, T. T. Chen, L. S. Chen, H. Cao, F. Yang and Y. L. Bai, CdTe quantum dots (QDs) improve the affinities of baicalein and genistein for human serum albumin in vitro, J. Inorg. Biochem., 2010, 104, 1148–1155 CrossRef CAS.
- J. B. Xiao, L. S. Chen, F. Yang, C. X. Liu and Y. L. Bai, Green, yellow and red emitting CdTe QDs decreased the affinities of apigenin and luteolin for human serum albumin in vitro, J. Hazard. Mater., 2010, 182, 696–703 CrossRef CAS.
- M. Idowu, E. Lamprecht and T. Nyokong, Interaction of water soluble thiol capped CdTe quantum dots and bovine serum albumin (BSA), J. Photochem. Photobiol., A, 2008, 198, 7–12 CrossRef CAS.
- F. J. Wang, J. Liu, Z. J. Wang, A. J. Lin, H. Luo and X. B. Yu, Interfacial heterostructure phenomena of highly luminescent ZnS/ZnO quantum dots, J. Electrochem. Soc., 2011, 158, H30–H34 CrossRef CAS.
- J. B. Xiao, H. Cao, Y. F. Wang, J. Y. Zhao and X. L. Wei, Glycosylation of dietary flavonoids decreases the affinities for plasma protein, J. Agric. Food Chem., 2009, 57, 6642–6648 CrossRef CAS.
- J. B. Xiao, M. Suzuki, X. Y. Jiang, X. Q. Chen, K. Yamamoto and M. Xu, Influence of B-ring hydroxylation on interactions of flavonols with bovine serum albumin, J. Agric. Food Chem., 2008, 56, 2350–2356 CrossRef CAS.
- J. B. Xiao, H. Cao, Y. F. Wang, K. Yamamoto and X. L. Wei, Structure-affinity relationship of flavones on binding to serum albumins: Effect of hydroxyl groups on ring A, Mol. Nutr. Food Res., 2010, 54, S253–S260 CAS.
- J. B. Xiao, H. Cao, T. T. Chen, F. Yang, C. X. Liu and X. C. Xu, Molecular property-binding affinity relationship of flavonoids for common rat plasma proteins in vitro, Biochimie, 2011, 93, 134–140 CrossRef CAS.
- U. Kragh-Hans, V. T. G. Chuang and M. Otagiri, Practical aspects of the ligand-binding and enzymatic properties of human serum albumin, Biol. Pharm. Bull., 2002, 25, 695–704 Search PubMed.
- J. M. Chow, S. C. Shen, S. K. Huan, H. Y. Lin and Y. C. Chen, Quercetin, but not rutin and quercitrin, prevention of H2O2-induced apoptosis via anti-oxidant activity and heme oxygenase 1 gene expression in macrophages, Biochem. Pharmacol., 2005, 69, 1839–1851 CrossRef CAS.
- H. Y. Lin, S. C. Shen and Y. C. Chen, Anti-inflammatory effect of heme oxygenase 1: Glycosylation and nitric oxide inhibition in macrophages, J. Cell. Physiol., 2005, 202, 579–590 CrossRef CAS.
-
I. Buxton, Pharmacokinetics and pharmacodynamics: the dynamics of drug absorption, distribution, action and elimination, in Goodman & Gilman's The Pharmacological Basis of Therapeutics (Brunton L ed), Chicago, 2005. pp 1–39 Search PubMed.
- J. Valanta, D. Drobne, K. Sepčić, A. Jemec, K. Kogej and R. Kostanjšek, Hazardous potential of manufactured nanoparticles identified by in vivo assay, J. Hazard. Mater., 2009, 171, 160–165 CrossRef.
- A. L. Di Virgilio, M. Reigosa, P. M. Arnal and M. F. L. de Mele, Comparative study of the cytotoxic and genotoxic effects of titanium oxide and aluminium oxide nanoparticles in Chinese hamster ovary (CHO-K1) cells, J. Hazard. Mater., 2010, 177, 711–718 CrossRef CAS.
- V. Shah, P. Dobiášová, P. Baldrian, F. Nerud, A. Kumar and S. Seal, Influence of iron and copper nanoparticle powder on the production of lignocellulose degrading enzymes in the fungus Trametes versicolor, J. Hazard. Mater., 2010, 178, 1141–1145 CrossRef CAS.
Footnotes |
† Electronic supplementary information (ESI) available. See DOI: 10.1039/c1an15457e |
‡ The same contribution as the first author. |
|
This journal is © The Royal Society of Chemistry 2012 |
Click here to see how this site uses Cookies. View our privacy policy here.