DOI:
10.1039/C2RA21037A
(Communication)
RSC Adv., 2012,
2, 9359-9361
Utilizing AgCl:Ag and AgCl mesostructures as solid precursors in the formation of highly textured silver nanomaterials via electron-beam induced decomposition†
Received
30th January 2012
, Accepted 7th August 2012
First published on 8th August 2012
Abstract
Herein, we report on the facile synthesis of AgCl:Ag and AgCl mesocubes with edge lengths up to 500 nm. When these mesostructures were placed under an electron beam, localized heating resulted in the decomposition of the AgCl regions of the respective materials leading to the formation of highly textured silver nanostructures. Furthermore, we utilized the AgCl:Ag and AgCl mesocubes along with their respective silver analogs for use in surface enhanced Raman spectroscopy (SERS) applications.
Introduction
Plasmonic materials are useful in a number of applications ranging from catalysis to SERS.1–3 In particular, meso and nano materials composed of silver or silver hybrid structures have gained significant research interest because of their unique plasmonic properties which can allow them to be employed across multiple fields of interest.4–6 Specifically, much attention has been directed to the synthesis of meso and nanostructures of silver hybrid materials composed of AgCl:Ag, resulting in a wide variety of morphologies ranging from particles to cubes.7–10 These novel AgCl:Ag materials are typically produced via the generation of silver halide precursors which are then placed under heat or in a dye solution and exposed to light thereby causing a partial reduction of the Ag(I) to Ag (0) yielding the desired hybrid composition. Moreover, these hybrid structures have been shown to be very useful as photocatalytic agents in a number of organic reactions with particular emphasis being given to their utilization in the photodecomposition of dyes. However, there does not appear to be any reported use of the plasmonic capabilities of these materials outside of the field of catalysis.
The utilization of an electron-beam to control the formation of nanostructures has recently gained prominence.11–13 One of the most widely employed methods of this type is the electron beam induced deposition (EBID) process, in which an unstable compound is exposed to the electron beam resulting in the controlled decomposition of the unstable compound at the site of exposure.12,13 The EBID process typically uses gases such as TiCl4, Fe(CO)5, or SiH4, more recently liquids have also been shown to be useful.14–17 The resultant nanoarchitectures are functional in such applications as catalysis, emission arrays and electronics. In order to expand the use of this novel process, further development is needed for the generation of solid-precursor materials. To this end, materials with silver halide components such as AgCl are excellent candidates for such a function as they are prone to rapid decomposition forming Ag(0) and Cl2 gas upon exposure to light or heat thereby producing silver nanostructures.
Herein, we report on a new methodology for the direct synthesis of AgCl:Ag or AgCl mesostructures, through the modification of a polyol reduction process involving the incorporation of high concentrations of either Rhodamine 6G (R6G) or HCl respectively. By utilizing this technique we produced cubic structures with edge-lengths up to 500 nm. When these materials were exposed to an electron beam they morphed into highly textured structures composed solely of silver as a result of the decomposition of AgCl regions in the precursor material. Finally we demonstrated the usefulness of these mesostructures as well as their silver analogs in SERS applications.
Results and discussion
Synthesis of AgCl:Ag and AgCl mesocubes
Fig. 1A displays the scanning electron microscope (SEM) image of the as-prepared AgCl:Ag cubic mesostructures. This was accomplished by adding a 208 mM solution of R6G to a standard silver nanocube synthesis, previously developed by Xia and coworkers.18 After 80 min of reaction, cubic structures with edge-lengths of ∼500 nm formed (Fig. 1A, inset). Furthermore, we could produce cubes of smaller edge-lengths by simply removing the reaction solution at earlier times (ESI† S2). Through the utilization of X-ray diffraction (XRD) we determined that these structures were composed of AgCl:Ag. Specifically, these mesocubes were produced via a simple precipitation route in which the R6G acted as a chloride source to form the AgCl components (ESI† S1, black). Moreover, as the AgCl mesocubes grow the R6G will further act as a slight reducing agent due to it being an electron donor causing the reduction of the AgCl regions to form Ag islands within and on the exterior of the mesocubes. Furthermore, the full reduction of AgCl was obstructed by the extreme excess of chloride in the reaction. To show that the reductive capabilities of the R6G dye were leading to the formation of the Ag regions, we substituted HCl for R6G in the reaction solution leaving ethylene glycol (EG) as the sole reducing agent. The resultant structures had similar shape and edge lengths to the samples prepared with R6G (Fig. 1B). However, when analyzed via XRD there was an absence of the peak at 38 2θ/deg which indicates that EG was not a strong enough reducing agent to from Ag(0) as these structures were composed solely of AgCl (ESI† S1, red).
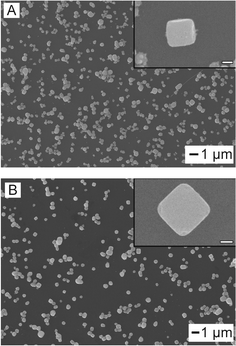 |
| Fig. 1 SEM images of the synthesized AgCl:Ag (A) and AgCl (B) cubic mesostructures. Inset scale bar is 200 nm. | |
Decomposition to form Ag nanostructures
Interestingly, when the mesocubes of AgCl:Ag or AgCl are exposed to the SEM electron beam (e-beam) they undergo a rapid morphological and compositional change. We first analyzed this affect on the AgCl:Ag particles shown in Fig. 2A where we see the initial cubic structure. Once the E-beam is focused on a single particle we begin to observe the morphology transformation, with ∼50 nm-sized features starting to form on the surface (Fig. 2B). Furthermore, when analyzed by energy-dispersive X-ray spectroscopy (EDAX) there is an immediate increase in the overall percentage of Ag in the mesocube (ESI† S3, black). The compositional transformation of the hybrid mesostructures to silver indicates that the e-beam is rapidly inducing the decomposition of the AgCl regions of the sample into Ag (0) and chlorine. The resultant Ag atoms are then nucleated on any of the silver islands within the mesocube structure and on its surface yielding the more textured morphology. As we increased the duration of e-beam exposure, the structure continued to change becoming more dendritic along with a further increase in the ratio of the Ag in the sample composition (Fig. 2C; ESI† S3, black). Fig. 2D shows that eventually there will be little change in our material's structure. Moreover, when these structures are analyzed with EDAX it is evident that Cl has almost entirely been removed from our system thereby creating dendritic nanostructures composed solely of Ag (ESI† S3, black). We then placed the AgCl mesostructures under the same conditions (Fig. 2E). Upon immediate e-beam exposure we did not notice as rapid a morphology change when compared to the AgCl:Ag sample with only small particles forming on the surface (Fig. 2F). Furthermore, there was a commensurate decrease in the rate of AgCl decomposition to Ag as shown by EDAX (ESI† S3, red). The difference in the morphology change between the two samples may be due to the AgCl mesostructure lacking Ag regions where the reduced Ag atoms can rapidly nucleate; as such the formation of these Ag areas occurs first. Moreover, as the exposure time under the e-beam increases a more textured surface results as the reduced Ag atoms would now have a place to nucleate more readily (Fig. 2G). When the morphology change ceased the resultant nanostructures did not appear to have as rough a surface as the AgCl:Ag sample, although it had almost entirely become silver in composition (ESI† S3, red).
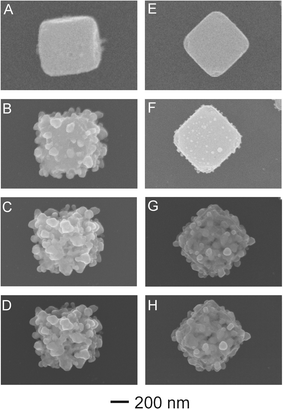 |
| Fig. 2 SEM images of a singular AgCl:Ag (A–D) and AgCl (E-H) mesostructures and their pathways to the formation of highly textured silver nanomaterials after direct exposure to the e-beam. | |
SERS capabilities of AgCl:Ag, AgCl, and their silver analogs
We then tested the SERS capabilities of the AgCl:Ag and AgCl cubes and compared them to their silver analogs. To do so we applied R6G onto the immobilized nanostructures, and analyzed the spectra in the region around wavenumber 1512 cm−1. For our purposes, the enhancement factor (EF) was calculated for all spectra using the equation below:
EF = ISERS/Ibulk × Nbulk/Nads |
Nbulk is the number of analyte molecules in the focal volume and Ibulk is the intensity of the Raman signal as calculated from the peak area of the 1512 cm−1 band for spectra taken of the analyte on a bare silicon substrate, while Nads and ISERS are the same parameters when the SERS substrate is utilized. We first analyzed the cubic structures composed of AgCl:Ag which yielded an EF of 9.35 × 103 (Fig. 3A, black). This enhancement factor most likely caused silver islands on the AgCl:Ag surface yielding the plasmonic features needed to enhance the Raman signal. After exposure of the AgCl:Ag mesostructures to the e-beam of a SEM we obtained an EF of 4.62 × 104 (Fig. 3A, red). This was a ∼5 fold increase in the intensity of the Raman signal and can be attributed to the change in the composition from AgCl:Ag to almost entirely silver. Also, the larger EF can be attributed to the dendritic features of the structure as each dendrons close proximity to one another led to a concentration of the electromagnetic field resulting in hot spot formation. We then turned our attention to materials composed of AgCl in which case our EF was 6.17 × 102 (Fig. 3B, black). In comparison to the AgCl:Ag we saw the order of magnitude drop in the EF due to a lack of exposed silver on the surface. When the Ag analog of the AgCl sample was analyzed the enhancement of the Raman signal increased by ∼7.7 fold to 4.74 × 103 (Fig. 3B, red). This result is less than the EFs obtained from the AgCl:Ag corresponding Ag counterpart, that was most likely caused by the resultant Ag morphology not being as highly textured as that of the e-beamed AgCl:Ag sample which yielded fewer hot spots on the surface.
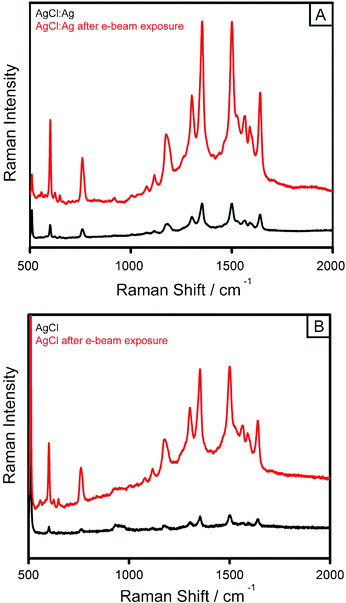 |
| Fig. 3 SERS spectra of Rhodamine 6G adsorbed onto AgCl:Ag and AgCl cubes along with their silver analogs: (A) AgCl:Ag cubes (black), and after e-beam induced formation of the Ag dendrite analog (red); (B) displays the same effect between the AgCl cube (black) and its corresponding Ag counterpart (red). The concentration of R6G is 0.1 mM. | |
Conclusions
In summary, we have developed a facile synthesis of AgCl:Ag cubic mesostructures via the incorporation of Rhodamine 6G acting as the chloride source into a polyol reduction reaction. Further, we demonstrated that high concentrations of HCl resulted in similar mesocubes composed of AgCl. When these materials were placed under an electron beam, the AgCl regions of the respective mesostructures decomposed to form highly textured silver nanomaterials. We studied the SERS capabilities of AgCl:Ag and AgCl cubes and their silver-dendrite analogs, proving them to have excellent plasmonic properties resulting in large enhancement factors of the Raman signal.
Acknowledgements
This research was conducted at the Center for Nanophase Materials Sciences, which is sponsored by the Oak Ridge National Laboratory by the Division of Scientific User Facilities, U.S. Department of Energy. The research was supported in part by the appointment of E.V. Formo and W. Fu to the ORNL Postdoctoral Research Associates Program, administered jointly by ORNL and the Oak Ridge Associated Universities. The Oak Ridge National Laboratory is managed by UT-Battelle, LLC for the U.S. Department of Energy under contract DE-AC05-00OR22725.
References
- P. K. Jain, X. Huang, I. E. El-Sayed and M. A. El-Sayed, Acc. Chem. Res., 2008, 41, 1578–1586 CrossRef CAS.
- W. H. Hung, M. Aykol, D. Valley, W. Hou and S. B. Cronin, Nano Lett., 2010, 10, 1314–1318 CrossRef CAS.
- J. P. Camden, J. A. Dieringer, J. Zhao and R. P. Van Duyne, Acc. Chem. Res., 2008, 41, 1653–1661 CrossRef CAS.
- P. K. Jain, X. Huang, I. H. El-Sayed and M. A. El-Sayed, Acc. Chem. Res., 2008, 41, 1578–1586 CrossRef CAS.
- J. Fang, B. Ding and H. Gleiter, Chem. Soc. Rev., 2011, 40, 5347–5360 RSC.
- M. Rycenga, C. M. Cobley, J. Zeng, W. Li, C. H. Moran, Q. Zhang, D. Qin and Y. Xia, Chem. Rev., 2011, 111, 3669–3712 CrossRef CAS.
- Z. Yan, G. Compagnini and D. B. J. Chrisey, J. Phys. Chem. C, 2011, 115, 5058–5062 CrossRef CAS.
- P. Wang, B. Huang, X. Qin, X. Zhang, Y. Dai, J. Wei and M. Whangbo, Angew. Chem., Int. Ed., 2008, 47, 7931–7933 CrossRef CAS.
- P. Wang, B. Huang, Z. Lou, X. Zhang, X. Qin, Y. Dai, Z. Zheng and X. Wang, Chem.–Eur. J., 2010, 16, 538–544 CrossRef CAS.
- C. An, S. Peng and Y. Sun, Adv. Mater., 2010, 22, 2570–2574 CrossRef CAS.
- M. A. Van Huis, A. Figuerola, C. Fang, A. Béché, H. W. Zandbergen and L. Manna, Nano Lett., 2011, 11, 4555–4561 Search PubMed.
- L. Serrano-Ramón, R. Córdoba, L. A. Rodríguez, C. Magén, E. Snoeck, C. Gatel, I. Serrano, M. R. Ibarra and J. M. De Teresa, ACS Nano, 2011, 5, 7781–7787 Search PubMed.
- I. Sychugov, Y. Nakayama and K. Mitsuishi, J. Phys. Chem. C, 2009, 113, 21516–21519 Search PubMed.
- S. H. Kim and G. A. Somorjai, J. Phys. Chem. B, 2002, 106, 1386–1391 CrossRef CAS.
- T. Lukasczyk, M. Schirmer, H. P. Steinrueck and H. Marbach, Langmuir, 2009, 25, 11930–11939 CrossRef CAS.
- M. G. Jenke, D. Lerose, C. Niederberger, J. Michler, S. Christiansen and I. Utke, Nano Lett., 2011, 11, 4213–4217 Search PubMed.
- E. U. Donev and J. T. Hastings, Nano Lett., 2009, 9, 2715–2718 CrossRef CAS.
- Q. Zhang, W. Li, L. Wen, J. Chen and Y. Xia, Chem.–Eur. J., 2010, 16, 10234–10239 CrossRef CAS.
Footnote |
† Electronic supplementary information (ESI) available. See DOI: 10.1039/c2ra21037a |
|
This journal is © The Royal Society of Chemistry 2012 |
Click here to see how this site uses Cookies. View our privacy policy here.