DOI:
10.1039/C2AN36407G
(Paper)
Analyst, 2013,
138, 299-306
3,5-Diformyl-borondipyrromethene for selective detection of cyanide anion†
Received
29th September 2012
, Accepted 24th October 2012
First published on 25th October 2012
Abstract
Cyanide is one of the most toxic inorganic anions, it is very harmful to human health but extremely useful in industrial activities. Herein, we used our recently reported boradiazaindacene (BODIPY) dye, 3,5-diformyl-borondipyrromethene (BODIPY 1) as an exclusive chemodosimetric and colorimetric sensor for CN− ion. Cyanide ion attacks the carbonyl groups of 1via a nucleophilic addition reaction and converts to cyanohydrin which is reflected in the clear colour change as well as by the absorption, emission and electrochemical properties. Thus BODIPY 1 can be used as a colorimetric and chemodosimetric sensor for CN− ion. Furthermore, to show that the position of the formyl group on BODIPY plays an important role in the ability of BODIPY dye to act as a chemodosimetric sensor for CN− ion, we synthesized another formyl group containing BODIPY dye, 3, in which the formyl group is present at the para-position of the meso-phenyl group. 1H NMR studies confirmed the formation of the cyanohydrin form of BODIPY dye 3 on addition of CN− ion but dye 3 cannot be used as a chemodosimetric sensor for CN− ion, as verified by absorption and fluorescence studies. The detection of cyanide with BODIPY dye 1 for biological application was also performed in MDA-MB-231 cells.
Introduction
Anions are ubiquitous in nature and in biological processes and they are also implicated in industrial and agricultural pollution.1 Hence, development of selective systems for their easy detection has received considerable attention in recent decades.2 Cyanide is one of the most toxic inorganic anions and is very harmful to the environment and human health.3 However, cyanide is extremely useful in the preparation of a wide variety of products ranging from plastics, fibres, gold, dyes, chelating agents for water treatment, to pharmaceuticals.4 Because of its widespread use, cyanide pollutes the environment and poses a severe threat to mammals since it is a potent inhibitor for some metallo-enzymes and non-metallo-enzymes.5 Consequently, there is a growing interest in sensing the presence of the toxic cyanide anion by a variety of fluorescent probes.6
Boradiazaindacene (BODIPY) dyes have received tremendous attention in recent years because of their excellent photophysical properties,7 such as long absorption and fluorescence wavelengths, high extinction coefficients, high fluorescence quantum yields, decent singlet state lifetimes and high photostability.8 Furthermore, the BODIPY dyes are easily amenable to structural modifications and the absorption and emission bands can be shifted at will by introducing the appropriate substituents.9 These dyes are widely used as biomolecular labels, chromogenic probes, drug delivery agents, fluorescent switches, electroluminescent films, laser dyes, light-harvesters and sensitizers for solar cells.10 BODIPY dyes have also been used extensively for metal cation sensors but there are only few reports on BODIPY-based anion sensors.11 Recently, we reported the synthesis of 3,5-diformyl-borondipyrromethene 1 (Chart 1) under very simple reaction conditions and its use as a pH sensor.12 The formyl groups at the 3,5-positions of the BODIPY core are very reactive and enable the preparation of several interesting dyes possessing novel properties. We prepared 3,5-bis(dipyrromethanyl)-borondipyrromethene 2 by treating 3,5-diformyl-BODIPY with pyrrole under mild acid catalyzed conditions and showed that 3,5-bis(dipyrromethanyl)-BODIPY 2 can be used as an exclusive sensor of fluoride ion (Chart 1).13 Herein we report one more interesting feature of 3,5-diformyl-BODIPY 1 that it can be used as a specific chemodosimeter and colorimetric sensor for cyanide anion by using spectral and electrochemical techniques. The two carbonyl groups at the 3- and 5-positions of BODIPY dye 1 are reactive sites where CN− attacks via nucleophilic addition to form cyanohydrin thereby altering the electronic properties of the dye and leading to clear changes in the colour and NMR, absorption, electrochemical and fluorescence properties. Furthermore, to show that the position of the aldehyde group(s) on the BODIPY dye play an important role enabling the dye to act as a CN− sensor, we synthesized an alternative BODIPY dye, 3, in which the aldehyde group is placed at the para-position of the meso-phenyl group. Our spectral and electrochemical studies showed that only dye 1 can act as a chemodosimeter and colorimetric sensor for cyanide anion.
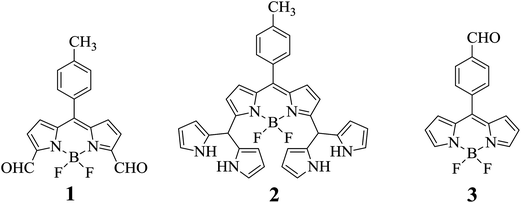 |
| Chart 1 Structures of BODIPYs 1, 2 and 3. | |
Results and discussion
BODIPY dye 1 was prepared as reported by us earlier.11 Dye 3 was prepared14 by a modified protocol shown in Scheme 1. Condensation of 4-hydroxymethyl benzaldehyde with excess pyrrole in CH2Cl2 under acid catalyzed conditions resulted in the formation of meso-(4-hydroxymethylphenyl) dipyrromethane 4. The dipyrromethane 4 was first oxidized with DDQ in CH2Cl2 for 1 h to afford meso-(4-hydroxymethylphenyl) dipyrromethene. Without isolating the dipyrromethene, the reaction mixture was first neutralized with triethylamine and then reacted with BF3·OEt2 at room temperature for 1 h. The crude compound was subjected to silica gel column chromatographic purification and afforded clean meso-(4-hydroxymethylphenyl) BODIPY 5. The alcohol group of meso-(4-hydroxymethylphenyl) BODIPY 5 was oxidized to aldehyde by treating 5 with activated MnO2 in CH2Cl2 at reflux temperature for 4 h followed by column chromatographic purification on silica and afforded pure 3 in 54% yield as an orange solid. Compound 3 was confirmed by HR-MS mass, 1H, 19F and 11B NMR techniques. In the 1H NMR spectrum, the six pyrrole protons appeared as three sets of signals in the 6.5–7.0 ppm region and the aldehyde proton appeared as a singlet at 10.15 ppm. Compound 3 showed a characteristic quartet at −145 ppm in 19F NMR and a characteristic triplet at 0.3 ppm in 11B NMR like any other meso-aryl BODIPYs.15
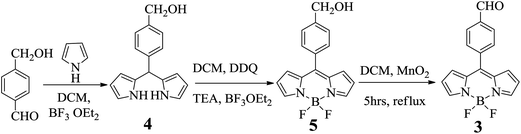 |
| Scheme 1 Synthesis of meso-(4-formyl phenyl) BODIPY 3. | |
Selective detection of cyanide anion by NMR, absorption, fluorescence and electrochemical studies by chemodosimetric approach
The chemodosimeter approach involves the use of specific chemical reactions induced by the presence of target anions that are coupled to a color or spectral variation.16 In this, the anion reacts with a chemodosimeter and catalyzes a chemical reaction. Since the final compound is chemically different to the original one, the spectroscopic characteristics of the compound will change, allowing for the determination of the anion. We used the chemodosimetric approach to detect cyanide anion using BODIPY dye 1. CN− ion reacts with carbonyl groups present at the 3,5-positions of the BODIPY core of 1via nucleophilic addition and converts to the cyanohydrin form which can be monitored by following changes in the absorption and fluorescence bands and redox potentials.
The conversion of the formyl group of BODIPY 1 to the cyanohydrin form by treating BODIPY 1 with CN− ion was followed systematically by 1H NMR titration. The incremental addition of tetra-n-butylammonium cyanide (TBACN) to a solution of BODIPY 1 in CD3CN is shown in Fig. 1. We used a maximum of two equivalents of TBACN which is required to react with two carbonyl groups present at the 3,5-positions of BODIPY 1 to convert them to the cyanohydrin forms. As is clear from Fig. 1, following sequential addition of CN− to compound 1, systematic downfield shifts were observed for pyrrole and meso-aryl protons. However, the most noticeable changes were observed for aldehyde proton Ha at 10.5 ppm in compound 1. On incremental addition of CN− to the solution of 1, the intensity of the sharp peak at 10.5 ppm corresponding to aldehyde proton (Ha) slowly decreased and at the same time a new signal at 4.2 ppm slowly appeared and increased in intensity corresponding to cyanohydrin –CH(CN)OH (Hb). Upon complete addition of two equivalents, the signal corresponding to aldehyde groups Ha completely disappeared and a signal corresponding to cyanohydrin Hb appeared supporting the conversion of compound 1 to the cyanohydrin form by attack of CN− ion. Similarly, the 1H NMR studies were also carried with compound 3 in which the formyl group is present at the para-position of meso-phenyl group. Following the addition of two equivalents of CN− to compound 3, the intensity of the sharp peak at 10.1 ppm corresponding to aldehyde proton (Hc) disappeared completely and at the same time a new signal at 5.4 ppm appeared corresponding to cyanohydrin –CH(CN)OH (Hd) as shown in Fig. 2. It is to be noted that in the case of BODIPY 3, when CN− ion reacts with the carbonyl group, it generates one chiral centre by the formation of cyanohydrin and the compound exists as a racemate. However, in the case of dye 1, reaction of CN− ion with the carbonyl groups present at the 3,5 positions of BODIPY generates two chiral centres by the formation of cyanohydrin and exists in meso and racemic mixtures. Thus, it is clear from the 1H NMR studies that aldehyde groups on the BODIPY unit are converted to the cyanohydrin form by the attack of CN− ion.
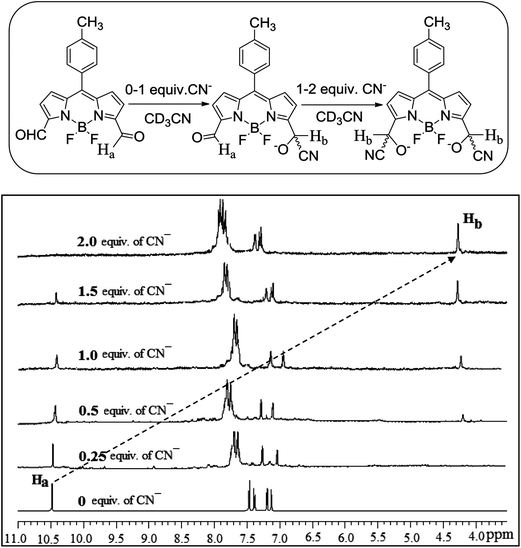 |
| Fig. 1 Partial 1H NMR spectra of compound 1 (2.2 × 10−2 M) in the presence of different concentrations of CN− ion in CD3CN. Concentration of CN− ion was varied from 0 to 2.0 equiv. | |
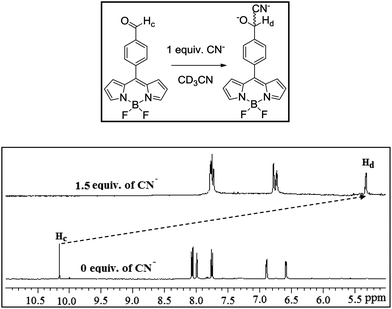 |
| Fig. 2 Partial 1H NMR spectrum of compound 3 (2.2 × 10−2 M) in the presence of 1.5 equivalents of CN− ion in CD3CN. | |
The conversion of the BODIPY aldehyde form to the BODIPY cyanohydrin form was systematically studied by absorption, fluorescence and electrochemical techniques. The BODIPY dyes, in general exhibit very interesting absorption and fluorescence properties and it is convenient to follow changes in absorption and emission peak maxima if there are any alterations in the electronic properties of the dye. The absorption spectra of BODIPY 1 showed a strong band corresponding to the S0 → S1 transition at 540 nm with one vibronic component on the higher energy side. In addition, an ill-defined band at 412 nm corresponding to a S0 → S2 transition is also present. Similarly, the absorption spectra of BODIPY 3 showed a strong band at 500 nm along with an ill-defined band at 350 nm. Thus, the absorption bands of dye 1 exhibit red shifts compared to dye 3. This is because the formyl groups are in direct conjugation with the BODIPY core in dye 1 and alter the electronic properties of the dye whereas the formyl group in dye 3 present at the para position of meso-phenyl group is not in direct conjugation with dye and doesn't alter the electronic properties of the dye. Thus, we carried out absorption spectral titration of compounds 1 and 3 with the increasing amounts of CN− in CH3CN. The systematic changes in the absorption spectra of compound 1 on addition of TBACN in CH3CN are shown in Fig. 3a. The addition of CN− to an acetonitrile solution of 1 resulted in a decrease of absorption bands at 540 and 412 nm and the appearance of new bands at 490 and 350 nm with three clear isosbestic points at 496, 450 and 370 nm supporting the formation of a cyanohydrin product. This is also clearly evident in the plot (inset of Fig. 3a) which shows the changes in the absorption band at 486 nm versus the amount of CN− ion. Importantly, the BODIPY dye 1 displays a very fast response (within 1 min) to cyanide at room temperature, and a maximal ratiometric absorption signal was achieved in the presence of only 2.2 equivalents of CN− ion. However, when the absorption titration was carried out with dye 3 in acetonitrile by addition of increasing amounts of CN− ion (Fig. S15†), although cyanohydrin formation occurs due to the attack of CN− ion on the carbonyl group present at the meso-phenyl group, there is a slight decrease in the intensity of the absorption band at 500 nm but there is no shift in the absorption peak maxima indicating that dye 3 cannot be used as a chemodosimetric sensor for CN− ion.
![Absorption spectra of compound 1 (5 × 10−6 M), (a) with different conc. of CN− (TBACN) solution (0–2.2 equiv.) in CH3CN. Inset: plot of A488/A538vs. [CN−]; (b) in presence of various anions (excess of equivalents) in CH3CN.](/image/article/2013/AN/c2an36407g/c2an36407g-f3.gif) |
| Fig. 3 Absorption spectra of compound 1 (5 × 10−6 M), (a) with different conc. of CN− (TBACN) solution (0–2.2 equiv.) in CH3CN. Inset: plot of A488/A538vs. [CN−]; (b) in presence of various anions (excess of equivalents) in CH3CN. | |
We also monitored the cyanide sensing phenomenon of dye 1 by fluorescence studies. Upon addition of cyanide anions, the fluorescence intensity of dye 1 at 554 nm decreased slowly and was completely quenched upon addition of two equivalents of cyanide anion in CH3CN as is shown in Fig. 4a. The significant change in the fluorescence intensity of compound 1 was noted upon addition of around 1.2 equiv of CN− ion, and the detection limit for CN− ion was 3 ppm under our experimental conditions. A Job plot analysis of the reaction of the sensor and CN− ions also corroborated the 1
:
2 binding stoichiometry (Fig. S14†). From the Benesi–Hildebrand equation, a binding constant of 2.7 × 104 M−1 was evaluated for the BODIPY 1–CN− anion complexation. We also evaluated the cyanide sensing phenomenon of dye 1 by fluorescence titration in aqueous CH3CN solution. Upon addition of cyanide ion to dye 1 in 3% aqueous acetonitrile solution (CH3CN
:
H2O; 97
:
3 v/v), the fluorescence intensity of dye 1 at 554 nm decreased (Fig. S17†) but was much slower than in pure CH3CN. Furthermore, it required nearly 15 equivalents of CN− in CH3CN
:
H2O (97
:
3 v/v) unlike in pure CH3CN in which it required only ∼2 equivalents of CN−. This result is in consistent with previously reported CN− ion probes, where the strong hydration of CN− ion in aqueous solution reduces its nucleophilicity.17 However, when we carried out titration in 1% aqueous solution (CH3CN
:
H2O; 99
:
1 v/v), the rate of fluorescence quenching was almost identical to that in pure CH3CN. As expected, the fluorescence spectrum of dye 3 did not show any major changes upon addition of increasing amounts of CN− in CH3CN (Fig. S15†). Furthermore, there were no changes in absorption and fluorescence bands of dye 1 upon addition of any other anions such as F−, Cl−, Br−, I−, HSO4−, H2PO4−, N3−, ClO4− as is shown in Fig. 3b and 4b. Thus, absorption and fluorescence studies clearly showed that dye 1 can act as a specific chromogenic and fluorescent chemodosimeter for cyanide anion whereas dye 3 cannot be used as a chemodosimetric sensor for CN− anion.
![Emission spectra of compound 1 (5 × 10−6 M), (a) upon titration with different conc. of CN−(TBACN) solution (0–2.2 equiv.) in CH3CN. The inset shows the plot of I/Imaxvs. [CN−]; (b) in presence of various anions (excess of equivalents) in CH3CN.](/image/article/2013/AN/c2an36407g/c2an36407g-f4.gif) |
| Fig. 4 Emission spectra of compound 1 (5 × 10−6 M), (a) upon titration with different conc. of CN−(TBACN) solution (0–2.2 equiv.) in CH3CN. The inset shows the plot of I/Imaxvs. [CN−]; (b) in presence of various anions (excess of equivalents) in CH3CN. | |
We further tested dye 1 for CN− sensing by following changes in the redox potentials of 1 upon addition of increasing amounts of CN− ion by square wave voltammetry. The systematic changes in the reduction waves of dye 1 upon increasing addition of CN− ion is shown in Fig. 5. The dye 1 showed two reversible reductions at −0.16 V and −1.15 V in CH3CN. Progressive addition of CN− to 1 resulted in a significant decrease of current intensity of reduction waves at −0.16 and −1.15 V and at the same time, the appearance of new reductions with a gradual increase in the current intensity at 0.10 and −0.72 V. Furthermore, no changes in reduction waves of 1 were observed upon addition of other anions such as F−, Cl−, Br−, I−, HSO4−, H2PO4−, N3−, ClO4−. Thus, the square wave voltammetric studies also supported the use of dye 1 as a specific electrochemical sensor for CN− ions.
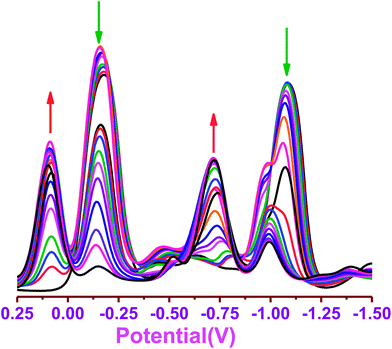 |
| Fig. 5 Square wave voltammogram of compound 1 (1.2 × 10−2 M) in the presence of different concentrations of CN− (TBACN) (0–2 equiv.). | |
Dye 1 can also be used as a colorimetric sensor as clear colour changes upon addition of CN− are visible under a UV lamp and to the naked eye. Addition of CN− ions to dye 1 resulted in a clear change in the colour of dye 1 from a bright green fluorescent colour to blue which was visible under a UV lamp (Fig. 6) and a colour change from orange to light yellow which was visible to the naked eye (Fig. 7). However, the addition of any other anions to dye 1 does not bring any noticeable colour change as shown in Fig. 6. Thus, dye 1 can be used exclusively as a chemodosimetric and colorimetric sensor for cyanide ion.
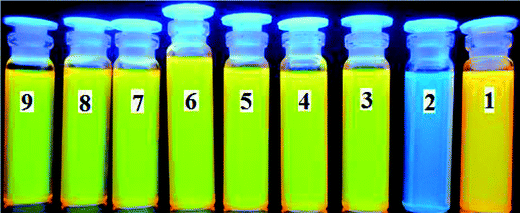 |
| Fig. 6 Color change induced upon addition of (excess equivalents of Bu4N+ salts) anions to receptor 1 (5 × 10−4 M in CH3CN) under UV lamp: from right to left: (1) no anion, (2) CN−, (3) F−, (4) Cl−, (5) Br−, (6) I−, (7) H2PO4−, (8) HSO4−, (9) ClO4−. | |
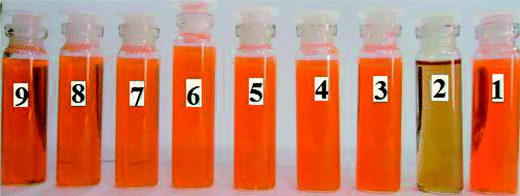 |
| Fig. 7 Color change induced upon addition of (excess equivalents of Bu4N+ salts) anions to receptor 1 (5 × 10−4 M in CH3CN) under daylight: from right to left: (1) no anion, (2) CN−, (3) F−, (4) Cl−, (5) Br−, (6) I−, (7) H2PO4−, (8) HSO4−, (9) ClO4−. | |
To test this sensor in a biological system, we used the human breast adenocarcinoma cell line MDA-MB-231. The cells were grown under standard conditions and treated with BODIPY dye 1 (100 μM), and at the end of 30 min (TBACN) CN− ion was added and cells were incubated for a further period of 30 min at 37 °C. The cells were washed twice with phosphate-buffered saline, and the fluorescence from living cells was observed using epifluorescence microscopy, both before and after the addition of CN− ion. The untreated cells did not show any fluorescence. Furthermore, persistence of cellular viabilities before and after treatment with CN− ion was observed from the bright field images As shown in Fig. 8, cells treated with the BODIPY dye 1 exhibited intense green emission (Fig. 8A) and the same showed a bleaching of green fluorescence when such cells were treated with CN− ion which is in agreement with the fluorescence studies.
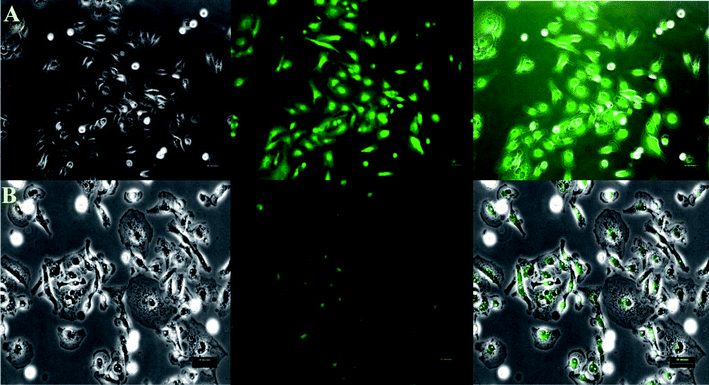 |
| Fig. 8 Live-cell imaging of MDA-MB-231 cells treated with BODIPY dye 1 before (A) and after (B) incubation with BODIPY dye 1 with CN− ion. The left panel represents the bright-field transmission images, the center panel represents the fluorescence and the right panel represents respective bright-field and overlay images. Scale 50 μm. | |
Conclusions
In summary, we used 3,5-diformyl-borondipyrromethene 1 as an exclusive chemodosimetric and colorimetric sensor for CN− ion. It is known that CN− ion reacts via nucleophilic addition reaction with the carbonyl group and converts it to cyanohydrin which we confirmed by NMR studies using BODIPY dye 1. Since the formyl groups are in direct conjugation with the BODIPY core in 1, their conversion to the cyanohydrin form alters the electronic properties of BODIPY which is clearly reflected by the colour change from yellow to blue which is visible under a UV lamp as well as by the changes in the absorption, emission and electrochemical properties. Thus, the BODIPY dye 1 can be used as a colorimetric and chemodosimetric sensor for CN− ion. However, when the formyl group is present on the meso-phenyl group of BODIPY dye 3, the conversion of the formyl group to cyanohydrin by CN− ion did not alter the electronic properties indicating that BODIPY 3 cannot be used for sensing CN− ion. Thus, the position of the formyl group plays an important role in designing BODIPY dye for chemodosimetric sensing of CN− ion. Furthermore, we have demonstrated that the BODIPY dye 1 can also be used in biological cell lines for the detection of CN− ion.
Experimental
General experimental
The chemicals such as BF3·Et2O, 2,3-dichloro-5,6-dicyano-1,4-benzoquinone (DDQ) were used as obtained from Aldrich. All other chemicals used for the synthesis were reagent grade unless otherwise specified. Column chromatography was performed on silica (60–120 mesh). The 1H, 13C, 11B and 19F NMR spectra were recorded in CDCl3 using a Bruker 400 MHz instrument and tetramethylsilane (Si(CH3)4) as the internal standard. Absorption and steady state fluorescence spectra were obtained with a Perkin-Elmer Lambda-35 and a PC1 photon counting spectrofluorometer manufactured by ISS, USA instruments, respectively. The elemental analyses were performed on a ThermoQuest microanalysis instrument. Square wave voltammetric (SWV) studies were carried out with a BAS electrochemical system utilizing the three electrode configuration consisting of a glassy carbon (working electrode), platinum wire (auxillary electrode) and saturated calomel (reference electrode) electrodes. The experiments were done in dry dichloromethane using 0.1 M tetrabutylammonium perchlorate (TBAP) as supporting electrolyte. The HR mass spectra were recorded with a Q-Tof micro mass spectrometer. For UV-vis and fluorescence titrations, the stock solution of compound 1 and 3 (5 × 10−6 M) was prepared by using spectroscopic grade CH3CN. The association constant of the anion complex formed in solution has been estimated by using the standard Benesi–Hildebrand equation, viz.,
Where I0 is the intensity of the compound 1 before addition of anion, I is the intensity in the presence of A−, I1 is intensity upon saturation with A−, and Ka is the association constant of the complex formed. The Bu4NCN (TBACN) solution was prepared (1 × 10−2 M) in CH3CN. The solution containing compound 1 and 3 was placed in a quartz cell (1 cm width), and Bu4NCN solution was added in an incremental fashion. Their corresponding UV-vis and fluorescence spectra were recorded at 298 K. For the 1H NMR titration, the spectra were measured using a 400 MHz NMR spectrometer. A solution of 1 and 3 in CD3CN was prepared (5 × 10−3 M), and a 0.4 mL portion of this solution was transferred to a 5 mm NMR tube. A small aliquot of Bu4NCN in CD3CN was added in an incremental fashion, and their corresponding spectra were recorded.
Biological studies
Materials.
Dulbecco's Modified Eagle's Medium (DMEM), fetal bovine serum (FBS), trypsin–EDTA in DPBS; 1% penicillin (50 U mL−1), and streptomycin (50 μg mL−1) were obtained from HiMedia Laboratories.
Cell culture.
MDA-MB-231 human breast adenocarcinoma cells were obtained from the National Centre for Cell science, Pune, India, and cultured on DMEM supplemented with 10% FBS, 1% penicillin (50 U mL−1) and streptomycin (50 μg mL−1) at 37 °C in 5% CO2 and 95% environment. Cells were seeded on six-well plates at a subconfluent density of 5000 cells per cm2 and stabilized overnight.
Incubation of cells with ligand and microscopic analysis.
For epifluorescene experiment, MDA-MB-231 human breast adenocarcinoma cells were incubated with BODIPY dye 1 at a concentration of 50–100 μM (in CH3CN; final volume less than 1% of total medium) and seeded onto six-well plates containing 3 mL of complete medium. The cells were incubated for 30 min at 37 °C in 5% CO2. After incubation, cells were washed twice with prewarmed (37 °C) phosphate-buffered saline (pH 7.4) and the cells were observed using epifluorescence microscopy (Olympus Ix71, Japan). The excitation wavelength used was 500 nm, and emission was measured at 554 nm through a green filter equipped with epifluorescence optics using a 10× phase-contrast objective, and a halogen lamp as a light source (Olympus, Japan). Images were taken with a Q imaging camera controlled by Image pro plus software.
General procedure for meso-(4-hydroxymethylphenyl) dipyrromethane (4).
BF3·Et2O (0.175 mmol) was added to a solution of corresponding 4-hydroxymethyl benzaldehyde (1.75 mmol) and pyrrole (70 mmol) in dichloromethane (100 mL) under a N2 atmosphere. The reaction mixture was stirred at room temperature for 1 h after that it was washed with 0.1 M NaOH solution and water thoroughly. The combined organic layers were dried over Na2SO4 and filtered. The pyrrole was evaporated under vacuum and the crude product was purified using silica gel column chromatography with petroleum ether (60–80 °C)–ethylacetate (80
:
20) and afforded pure meso-(4-hydroxymethylphenyl) dipyrromethane 4 as a white solid. Yield: 75%. 1H NMR (400 MHz, CDCl3, δ in ppm): 4.58 (s, 2H, –CH2OH), 5.41 (s, 1H, meso-CH), 5.86 (d, 3J (H, H) = 3.97 Hz, 2H, py), 6.13 (m, 2H, py), 6.63 (d, 3J (H, H) = 4.21 Hz, 2H, py), 7.17 (d, 3J (H, H) = 8.10 Hz, 2H, Ar), 7.24 (d, 3J (H, H) = 7.31 Hz, 2H, Ar), 8.0 (br s, 2H, NH). 13C NMR (100 MHz, CDCl3, δ in ppm): 43.8, 65.1, 107.3, 108.4, 117.5, 127.5, 128.7, 132.6, 139.5, 141.8. HRMS calcd for C16H16N2O [(M + 1)+]: m/z 253.1341. Found: m/z 253.1330. Anal. calcd for C16H16N2O: C, 76.16; H, 6.39; N, 11.10. Found: C, 76.09; H, 6.21; N, 11.33%.
4,4-Difluoro-8-(4-hydroxymethylphenyl)-4-bora-3a,4a-diaza-s-indacene (5).
meso-(4-Hydroxymethylphenyl) dipyrromethane 4 (1.7 mmol) was dissolved in dichloromethane (100 mL), and oxidized with DDQ (2.04 mmol) at room temperature. The reaction mixture was allowed to stir at room temperature for 30 min, followed by the addition of triethylamine (68 mmol). After 30 min, BF3·Et2O (85 mmol) was added, and the mixture was continuously stirred at room temperature for an additional 1 h. The reaction mixture was evaporated and the crude product was purified using silica gel column chromatography with petroleum ether–ethylacetate (50
:
50) and afforded pure meso-(4-hydroxymethylphenyl)-BODIPY 5 as an orange solid. Yield: 32%. 1H NMR (400 MHz, CDCl3, δ in ppm): 4.52 (s, 2H, –CH2OH), 6.15 (d, 3J (H, H) = 4.32 Hz, 2H, py), 6.62 (m, 2H, py), 8.23 (br s, 2H, py), 7.18 (d, 3J (H, H) = 7.82 Hz, 2H, Ar), 7.23 (d, 3J (H, H) = 8.55 Hz, 2H, Ar). 11B NMR (128.3 MHz, CDCl3, δ in ppm): 0.36 (t, 1J (B–F) = 32.5 MHz, 1B). 19F NMR (376.4 MHz, CDCl3, δ in ppm): –144.6 (q, 1J (F–B) = 35 MHz, 2F). 13C NMR (100 MHz, CDCl3, δ in ppm): 43.8, 64.5, 107.2, 108.3, 117.4, 118.7, 126.8, 128.7, 131.7, 132.5, 144.2. HRMS calcd for C16H13BF2N2O [(M − F)+]: m/z 279.1105. Found: m/z 279.1098. Anal. calcd for C16H13BF2N2O: C, 68.85; H, 4.69; N, 10.04. Found: C, 68.76; H, 4.61; N, 10.12%.
4,4-Difluoro-8-(4-formylphenyl)-4-bora-3a,4a-diaza-s-indacene (3).
To a solution of meso-(4-hydroxymethylphenyl)-BODIPY 5 (1.7 mmol) in dichloromethane (50 mL), activated MnO2 (42.5 mmol) was added and the mixture was stirred at room temperature until the consumption of alcohol was completed (4 h, as monitored by TLC). The mixture was filtered through Celite, the filtrate was evaporated, and then the solid residue was further purified using silica gel column chromatography with petroleum ether–ethylacetate (75
:
25) and afforded pure meso-(4-formylphenyl)-BODIPY 3 as a red solid. Yield: 56%. 1H NMR (400 MHz, CDCl3, δ in ppm): 6.58 (d, 3J (H, H) = 3.96 Hz, 2H, py), 6.88 (d, 3J (H, H) = 4.21 Hz, 2H, py), 7.74 (d, 3J (H, H) = 8.12 Hz, 2H, Ar), 8.0 (s, 2H, py), 8.05 (d, 3J (H, H) = 8.24 Hz, 2H, Ar). 10.15 (brs, 1H, –CHO). 11B NMR (128.3 MHz, CDCl3, δ in ppm): 0.31 (t, 1J (B–F) = 38 MHz, 1B). 19F NMR (376.4 MHz, CDCl3, δ in ppm): −144.8 (q, 1J (F–B) = 33.5 MHz, 2F). 13C NMR (100 MHz, CDCl3, δ in ppm): 29.9, 119.3, 129.7, 131.2, 131.5, 134.8, 137.8, 139.5, 145.3, 191.5. HRMS calcd for C16H11BF2N2O [(M − F)+]: m/z 277.0948. Found: m/z 277.0954. Anal. calcd for C16H11BF2N2O: C, 69.36; H, 4.00; N, 10.11. Found: C, 69.25; H, 4.11; N, 10.18%.
Acknowledgements
M.R. acknowledges financial support from the Board of Research in Nuclear Sciences (BRNS) and S.M. acknowledges IIT-Bombay for a fellowship.
Notes and references
-
(a) E. B. Veale and T. Gunnlaugsson, Annu. Rep. Prog. Chem., Sect. B: Org. Chem., 2010, 106, 376 RSC;
(b) P. D. Beer and P. A. Gale, Angew. Chem., Int. Ed., 2001, 40, 486 CrossRef CAS;
(c) V. Amendola, D. Esteban-Gómez, L. Fabbrizzi and M. Licchelli, Acc. Chem. Res., 2006, 39, 343 CrossRef CAS;
(d) S. K. Kim, J. H. Bok, R. A. Bartsch, J. Y. Lee and J. S. Kim, Org. Lett., 2005, 7, 4839 CrossRef CAS;
(e) J. S. Kim and D. T. Quang, Chem. Rev., 2007, 107, 3780 CrossRef CAS;
(f) H. J. Kim, S. Bhuniya, R. K. Mahajan, R. Puri, H. Liu, K. C. Ko, J. Y. Lee and J. S. Kim, Chem.Commun., 2009, 7128 RSC.
-
(a) C. Caltagirone and P. A. Gale, Chem. Soc. Rev., 2009, 38, 520 RSC;
(b) P. A. Gale, S. E. Garcia-Garrido and J. Garric, Chem. Soc. Rev., 2008, 37, 151 RSC;
(c) C. Suksai and T. Tuntulani, Chem. Soc. Rev., 2003, 32, 192 RSC;
(d) P. D. Beer and E. J. Hayes, Coord. Chem. Rev., 2003, 240, 167 CrossRef CAS;
(e) J. L. Sessler, S. Camiolo and P. A. Gale, Coord. Chem. Rev., 2003, 240, 17 CrossRef CAS;
(f) J. L. Sessler and J. M. Davis, Acc. Chem. Res., 2001, 34, 989 CrossRef CAS;
(g) S. L. Wiskur, H. Ait-Haddou, J. J. Lavigne and E. V. Anslyn, Acc. Chem. Res., 2001, 34, 963 CrossRef CAS;
(h) A. P. de Silva, H. Q. N. Gunaratne, T. Gunnlaugsson, A. J. M. Huxley, C. P. McCoy, J. T. Rademacher and T. E. Rice, Chem. Rev., 1997, 97, 1515 CrossRef CAS.
-
(a)
K. W. Kulig, Cyanide Toxicity, U.S. Department of Health and Human Services, Atlanta, 1991 Search PubMed;
(b)
S. I. Baskin and T. G. Brewer, Medical Aspects of Chemical and Biological Warfare, ed. F. Sidell, E. T. Takafuji and D. R. Franz, TMM Publication, Washington, 1997, ch. 10, p. 271 Search PubMed.
-
(a)
P. A. Patnaik, A Comprehensive Guide to the Hazardous Properties of Chemical Substances, van Nostrand Reinhold, New York, 1992, p. 229 Search PubMed;
(b)
G. J. Hathaway and N. H. Proctor, Chemical Hazards of the Workplace, John Wiley & Sons, Inc., Hoboken, 5th edn, 2004, p. 190 Search PubMed;
(c)
V. N. David, R. G. Luthy and G. M. Wong-Chang, Cyanide in Water and Soil: Chemistry, Risk and Management, CRC Press, 2005, ch. 4, p. 41 Search PubMed;
(d) M. A. Acheampong, R. J. W. Meulepasa and P. N. L. Lens, J. Chem. Technol. Biotechnol., 2010, 85, 590 CrossRef CAS;
(e)
C. Young, L. Tidwel and C. Anderson, Cyanide: Social, Industrial and Economic Aspects, Minerals, Metals, and Materials Society, Warrendale, PA, 2001, p. 35 Search PubMed.
- Z. Xu, X. Chen, H. N. Kim and J. Yoon, Chem. Soc. Rev., 2010, 39, 127 RSC.
-
(a) S.-H. Kim, S.-J. Hong, J. Yoo, S. K. Kim, J. L. Sessler and C.-H. Lee, Org. Lett., 2009, 16, 3626 CrossRef;
(b) L. Yuan, W. Lin, Y. Yang, J. Song and J. Wang, Org. Lett., 2011, 14, 3730 CrossRef;
(c) Z. Liu, X. Wang, Z. Yang and W. He, J. Org. Chem., 2011, 76, 10286 CrossRef CAS;
(d) S. Khantua, D. Samanta, J. W. Bats and M. Schmittel, Inorg. Chem., 2012, 51, 7075 CrossRef;
(e) K.-S. Lee, H.-J. Kim, G.-H. Kim, I. Shin and J.-I. Hong, Org. Lett., 2008, 10, 49 CrossRef CAS;
(f) S.-J. Hong, J. Yoo, S.-H. Kim, J. S. Kim, J. Yoon and C.-H. Lee, Chem. Commun., 2009, 189 RSC;
(g) J. O. Huh, Y. Do and M. H. Lee, Organometallics, 2008, 27, 1022 CrossRef CAS.
-
(a) A. Loudet and K. Burgess, Chem. Rev., 2007, 107, 4891 CrossRef CAS;
(b) R. Ziessel, G. Ulrich and A. Harriman, New J. Chem., 2007, 31, 496 RSC;
(c) N. Boens, V. Leen and W. Dehaen, Chem. Soc. Rev., 2012, 41, 1130 RSC.
-
(a) J.-H. Olivier, A. Haefele, P. Retailleau and R. Ziessel, Org. Lett., 2010, 12, 408 CrossRef CAS;
(b) A. B. Nepomnyashchii, S. Cho, P. J. Rossky and A. J. Bard, J. Am. Chem. Soc., 2010, 132, 17550 CrossRef CAS;
(c) K.-C. Antonia, N. Karakostas, C. P. Raptopoulou, V. Psycharis, E. Saridakis, J. Griebel, R. Hermann and G. Pistolis, J. Am. Chem. Soc., 2010, 132, 16327 CrossRef;
(d) J. Murtagh, D. O. Frimannsson and D. F. O'Shea, Org. Lett., 2009, 11, 5386 CrossRef CAS;
(e) S. Rihn, M. Erdem, A. D. Nicola, P. Retailleau and R. Ziessel, Org. Lett., 2011, 13, 916 CrossRef;
(f) A. Palma, L. A. Alvarez, D. Scholz, D. O. Frimannsson, M. Grossi, S. J. Quinn and D. F. O'Shea, J. Am. Chem. Soc., 2011, 133, 19618 CrossRef CAS;
(g) H. He, P.-C. Lo, S.-L. Yeung, W.-P. Fong and D. K. P. Ng, Chem. Commun., 2011, 47, 4748 RSC.
-
(a) O. Buyukcakir, O. A. Bozdemir, S. Kolemen, S. Erbas and E. U. Akkaya, Org. Lett., 2009, 11, 4644 CrossRef CAS;
(b) H. Sunahara, Y. Urano, H. Kojima and T. Nagano, J. Am. Chem. Soc., 2007, 127, 5597 CrossRef;
(c) Y. Cakmak and E. U. Akkaya, Org. Lett., 2009, 11, 85 CrossRef CAS;
(d) C. Thivierge, A. Loudet and K. Burgess, Macromolecules, 2011, 44, 4012 CrossRef CAS.
-
(a) R. Ziessel and A. Harrimon, Chem. Commun., 2011, 47, 611 RSC;
(b) A. Harrimon, L. J. Mallon, S. Goeb, G. Ulrich and R. Ziessel, Chem.–Eur. J., 2009, 15, 4553 CrossRef;
(c) T. Bura, R. Retailleau and R. Ziessel, Angew. Chem., Int. Ed., 2010, 49, 6659 CrossRef CAS;
(d) A. Harriman, G. Izzet and R. Ziessel, J. Am. Chem. Soc., 2006, 128, 10868 CrossRef CAS.
-
(a) X. Qi, E. J. Jun, L. Xu, S.-J. Kim, J. S. J. Hong, Y. J. Yoon and J. Yoon, J. Org. Chem., 2006, 71, 2881 CrossRef CAS;
(b) T. Cheng, Y. Xu, S. Zhang, W. Zhu, X. Qian and L. Duan, J. Am. Chem. Soc., 2008, 130, 16160 CrossRef CAS;
(c) S. C. Dodani, Q. He and C. J. Chang, J. Am. Chem. Soc., 2009, 131, 18020 CrossRef CAS;
(d) D. W. Domaille, L. Zeng and C. J. Chang, J. Am. Chem. Soc., 2010, 132, 1194 CrossRef CAS;
(e) L. Zeng, E. W. Miller, A. Pralle, E. Y. Isacoff and C. J. Chang, J. Am. Chem. Soc., 2006, 128, 10 CrossRef CAS;
(f) M. Yuan, Y. Li, J. Li, C. Li, X. Liu, J. Xu, H. Liu, S. Wang and D. Zhu, Org. Lett., 2007, 9, 2313 CrossRef CAS;
(g) S. Atilgan, T. Ozdemir and E. U. Akkaya, Org. Lett., 2008, 10, 4065 CrossRef CAS;
(h) R. Guliyev, S. Ozturk, E. Sahin and E. U. Akkaya, Org. Lett., 2012, 14, 1528 CrossRef CAS.
- S. Madhu, M. Rajeswararao, M. S. Shaikh and M. Ravikanth, Inorg. Chem., 2011, 50, 4392 CrossRef CAS.
- S. Madhu and M. Ravikanth, Inorg. Chem., 2012, 51, 4285 CrossRef CAS.
-
(a) N. M. Loim and E. S. Kelbyscheva, Russ. Chem. Bull., Int. Ed., 2004, 53, 2080 CrossRef CAS;
(b) T. Kálai and K. Hideg, Tetrahedron, 2006, 62, 10352 CrossRef.
-
(a) H. L. Kee, C. Kirmaier, L. Yu, P. Thamyongkit, W. J. Youngblood, M. E. Calder, L. Ramos, B. C. Noll, D. F. Bocian, W. R. Scheidt, R. R. Birge, J. S. Lindsey and D. Holten, J. Phys. Chem. B, 2005, 109, 20433 CrossRef CAS;
(b) A. Cui, X. Peng, J. Fan, X. Chen, Y. Wu and B. Guo, J. Photochem. Photobiol., A, 2007, 186, 85 CrossRef CAS;
(c) V. Leen, V. Z. Gonzalvo, W. M. Deborggraeve, N. Boens and W. Dehaen, Chem. Commun., 2010, 46, 4908 RSC.
-
(a) R. Martínez-Máńez and F. Sancenón, Chem. Rev., 2003, 103, 4419 CrossRef;
(b) X. Lou, D. Ou, Q. Li and Z. Li, Chem. Commun., 2012, 48, 8462 RSC;
(c) C. Zhao, P. Feng, J. Cao, X. Wang, Y. Yang, Y. Zhang, J. Zhang and Y. Zhang, Org. Biomol. Chem., 2012, 10, 3104 RSC.
- D.-S. Kim, Y.-M. Chung, M. Jun and K. H. Ahn, J. Org. Chem., 2009, 74, 4849 CrossRef CAS.
Footnote |
† Electronic supplementary information (ESI) available. See DOI: 10.1039/c2an36407g |
|
This journal is © The Royal Society of Chemistry 2013 |
Click here to see how this site uses Cookies. View our privacy policy here.