DOI:
10.1039/C3BM00167A
(Paper)
Biomater. Sci., 2013,
1, 519-527
Viscoelastic hydrogels from poly(vinyl alcohol)–Fe(III) complex†
Received
7th November 2012
, Accepted 4th February 2013
First published on 25th February 2013
Abstract
Hydrogels are three dimensional scaffolds of hydrophilic polymers with inter-connected water channels. In this investigation, an interesting approach for the fabrication of a highly viscoelastic hydrogel derived from a polyvinyl alcohol (PVA) based macromer is reported. PVA was crosslinked using Fe3+ ions under basic conditions to obtain a stable hydrogel with a highly porous network structure. The swelling ratio of the designed hydrogels was evaluated and correlated to the network structure of the metal coordinated crosslinked macromers. Compressive modulus and dynamic viscoelastic measurements were carried out using a dynamic mechanical analyzer and no significant changes in storage modulus were observed by increasing the temperature up to 45 °C. A creep study revealed that the elastic recovery was enhanced with an increase in the degree of crosslinking. The effect of crosslinking influenced properties such as the glass transition, melting point and melting enthalpy of the crosslinked network. The cytocompatibility of the gels was studied using human lung fibroblasts (IMR-90) and no toxic effects or significant cell attachments were observed on the surface of the gel after 5 days. The designed hydrogels may be useful for biomedical procedures which require highly viscoelastic, biocompatible, thermally and mechanically stable biomaterials.
Introduction
Design, synthesis and characterization of materials are important steps for developing a new class of bio-scaffold. Hydrogels with a water enriched network structure1 are suitable for applications such as matrices for the controlled release of pharmaceutical agents, contact lenses and bio-adhesives.2–7 Polymer based hydrogels have been prepared using different crosslinking methods such as chemical, physical and ionic crosslinking.8
Recently, various hydrophilic polymers have been used for preparing scaffolds for potential biomedical applications.9–11 The porous network structure of the gels favours the exchange of nutrients and waste products with the surrounding environment making them attractive substitutes for soft tissues12–14 and drug delivery applications.15,16 PVA gels are known to be biocompatible and stable up to 37 °C, at the same time, high hydrophilicity ensures minimal cell adhesion and protein adsorption, which are beneficial in biomedical applications.17–19 PVA hydrogels have shown interesting mechanical responses for developing porcine aortic tissue,20 human carotid arteries and for potential heart valve applications, artificial articular cartilages21,22 and for intravascular applications.23
PVA is a semi-crystalline polymer with pendent hydroxyl groups which can be further functionalized with monomers for synthesising functional hydrogels.24 PVA hydrogels have been formed via both chemical and physical crosslinking mechanisms.25
Similarly the adjacent hydroxyl groups of PVA can be crosslinked with glutaraldehyde under acidic conditions to form a highly crosslinked network.7,26,27 However, trace residual amounts of glutaraldehyde are known to be cytotoxic in nature. Photo-crosslinkable PVA hydrogels have been designed to remove any leachable toxic by-products and have been used in tissue engineering, drug delivery and other advanced biomedical applications.28–31
Recent research has focused on the development of various hydrogel scaffolds for applications ranging from nucleus pulposus replacement, vocal cord mucosa replacement, contact lenses and targeted drug delivery applications.32–35 All these scaffolds need strict compliance and high viscoelastic properties which impart low mechanical irritation towards surrounding tissues. However, the majority of the designed scaffolding materials have failed during dynamic applications in soft tissue substitutes due to a mismatch in the viscoelastic properties and stability. Hence developing hydrogel scaffolds with tunable physico-mechanical properties is challenging.
In this study, we develop highly viscoelastic PVA hydrogels by using environmentally friendly and nontoxic iron ions. Although a plethora of crosslinking techniques have been investigated to obtain hydrogels from PVA, non-toxic iron based crosslinking offers an interesting method to develop hydrogels for biomedical applications. Here, the physico-mechanical behaviour, cytotoxicity, cell adhesion and proliferation on PVA–Fe hydrogels are investigated in detail.
Materials and methods
Materials
PVA with a weight average molecular weight of 146
000–186
000 was purchased from Sigma Aldrich. FeCl3·6H2O was purchased from Biolab Int Ltd and NH4OH was purchased from Mallinckrodt Baker. The dialysis membrane (molecular weight cut off 3500) was purchased from Thermo Scientific and prepared according to an established procedure. Tissue culture polystyrene (TCPS) plates were purchased from Griener Bio-One. Dulbecco’s modified Eagle’s medium (DMEM) was purchased from PAA Laboratories. Phosphate buffered saline (PBS) was purchased from 1st BASE. All other chemicals used for the study were analytical grade.
Preparation of hydrogels
PVA solution (10 wt%) was prepared by heating PVA granules at 80 °C in Millipore water with constant stirring for 2 h. FeCl3 crystals were dissolved in Millipore water to prepare standard solutions of concentrations 0.1 M, 0.15 M, 0.2 M, and 0.25 M. Aliquots (1 ml) of these standard solutions were added to a 10% PVA solution (5 ml) kept in different vials, and mixed properly using a magnetic stirrer. To the above solution, NH4OH (1 ml) was added drop wise with continuous stirring for the formation of the hydrogel. Four different designed concentrations of FeCl3 were used to prepare the samples which are represented as PVFeGel-1, PVFeGel-2, PVFeGel-3 and PVFeGel-4, respectively. The mixing of PVA with other ions such as alkali and alkaline earth metal cations did not lead to gel formation.
Swelling study
To the hydrogel samples, a 20-fold excess amount of PBS solution (commonly used for biological studies) was added and the hydrogels were allowed to swell in an incubator at 37 °C. The surface of the hydrogel was swabbed with the help of blotting paper to remove excess PBS and the hydrogels were weighed in an analytical balance at regular time intervals to monitor the water absorption capacity inside the hydrogel networks. Experiments were done in triplicates. The swelling ratio (Qm) was calculated from the ratio of the weight of the PBS uptake to the weight of the dried gel.
where Ws is the total weight of the swollen hydrogel and Wd is the weight of the hydrogel dried by lyophilizer.
Furthermore, the effect of common electrolytes, such as K+, Na+ and Ca2+, towards the swelling behaviour was observed up to 5 days at 37 °C.
The swollen gel was dried using the freeze–dry method and the samples were cut carefully by a scalpel blade. The sample was coated with platinum and the resultant dried gel morphology was imaged using a JEOL JSM-6701F field emission scanning electron micrograph (Tokyo, Japan). The water swollen hydrogel was viewed under an optical microscope (Olympus, BX61). All FTIR measurements were carried out in attenuated total reflectance (ATR) mode (Thermo Scientific). The recording spectral range was fixed at 600–4000 cm−1 and the spectrum was evaluated using Ominic software.
Physico-mechanical behaviour of the hydrogels
Mechanical characterization.
The mechanical property measurements were carried out using a dynamic mechanical analyzer, DMA Q800 (TA instruments). All experiments were carried out in compression mode. PVA hydrogels were completely immersed in PBS solution and allowed to swell in an incubator at 37 °C. Moulded circular shaped samples measuring 10 mm in diameter and 2 to 3 mm in thickness were prepared using a die. DMA controlled force mode was used with a preload force of 0.01 N to measure the stress–strain property of the hydrogels. The temperature of the chamber was kept constant at 37 °C during force ramping at a rate of 0.1 N min−1 and the resulting mechanical properties were recorded.
For dynamic mechanical analysis, multifrequency-strain mode was used with a static load of 0.001 N and constant oscillating amplitude of 50. To study the influence on viscoelastic behaviour with change in frequency, the specimen was held isothermally at 37 °C and deformed at constant amplitude over a range of frequencies, 1 Hz to 10 Hz, and the mechanical properties were measured. To evaluate the change in the viscoelastic properties by changing the temperature, the specimens were exposed to a series of isothermal temperatures from 20 °C to 45 °C. At each temperature, the material was deformed at constant amplitude (strain 1%) over a frequency of 1 Hz and the subsequent mechanical properties were evaluated.
For the creep experiment, a constant static force of 0.01 N was applied and the temperature was kept at 37 °C throughout the experiment. A stress of 200 Pa was applied to the swollen hydrogel for 5 minutes and the hydrogel was allowed to relax for 10 minutes and the strain release from the sample was measured. From this the percentage strain developed and the strain recovery was calculated with time.
Cell culture.
Moulded shaped (3 mm diameter and 2 mm height) swollen gel samples were thoroughly washed with water, followed by PBS, sterilized under UV-irradiation and placed on the bottom of a 24-well culture plate. The gels were washed and conditioned in sterile PBS before seeding the cells. IMR-90 was purchased from Coriell Cell Repositories, USA, and maintained in modified Eagle’s medium with glutamine (MEM, PAA Laboratories), supplemented with 15% FBS (standard quality, EU-approved origin, PAA Laboratories GmbH) and 1% each of penicillin, streptomycin, non-essential amino acids, vitamins and 2% essential amino acids (Gibco, Invitrogen). Cells were subcultured at 80–90% confluence and maintained at 37 °C in a humidified incubator with 5% CO2.
Cell viability assay.
The viability of the cells on the respective hydrogels was measured using the CellTiter-Glo luminescent cell viability assay (Promega) following the manufacturer's instructions. This assay is a homogeneous method for determining the number of viable, metabolically-active cells in culture based on the quantification of the ATP concentrations. The procedure involves the addition of an equal volume of reagent to the medium in test wells, which in a single step generates a luminescent signal proportional to the concentration of the ATP present in the cells. The reagent contains detergents to break the cell membrane, causing ATP release into the medium and ATPase inhibitors to stabilize the released ATP. The assay is based on the conversion of beetle luciferin to oxyluciferin by a recombinant luciferase in the presence of ATP. For the ATP assay, ∼5000 cells were plated per well in a 24-well plate (Griener Bio-one) and the designed gel samples of 3 mm diameter and 2–3 mm height were placed above the cell monolayer. This was maintained for 1, 2 and 5 days in 1 ml medium at 37 °C. Fresh media was replaced every other day. At respective times, the medium in each well was removed and replaced with 200 μl fresh medium. CellTiter-Glo reagent (200 μl) pre-warmed to room temperature was added and mixed properly. After 10 min, the mixture was transferred to flat-bottom 96-well plates (Corning, Costar). Luminescence readings were measured using a Tecan Infinite F200 micro-plate reader.
Cell adhesion study.
IMR-90 cells were trypsinized using a trypsin–EDTA mixture to get cells in suspension. Approximately 5000 cells per vial were added on top of the swollen gel. Cells were allowed to adhere on to the material for 2 hours and then sufficient medium was added and incubated at 37 °C. Cell growth was monitored periodically on top of the hydrogel using an optical microscope (Olympus, BX61).
Results and discussion
PVA is an uncharged, water soluble polymer which is commercially prepared from the polymerization of vinyl acetate, followed by hydrolysis. PVA was selected for our study owing to its hydrophilic nature and the potential of chemically modifying the free pendent hydroxyl groups on the polymer backbone.36–39 In our investigation, a novel crosslinking method was used for the in situ gelation of the aqueous PVA solution by FeCl3 in basic medium. The crosslinking is due to the coordination of Fe(III) ions with the pendent hydroxyl groups of the PVA macromer. The camera images of the crosslinked gels are shown in the ESI (SI-1†). The Fe(III) ion is a high spin d5 species and generally forms hexacoordinate complexes with suitable ligands.40 In the coordination process, the replacement of Cl− is dependent on the pH of the medium. In basic medium, Cl− was replaced by –OH groups to form FeO(OH). Particularly, an increase in pH leads to the hydrolysis of the hydrated ions, forming hydroxo- and oxo-polynuclear complexes.41 To study the coordination behaviour of Fe(III) and PVA, infrared spectroscopy was used. After coordination with Fe(III) ions, the material became insoluble in most common solvents and ATR-FTIR was used to detect the interaction between the –OH groups of the PVA and the Fe(III) cations. The recorded IR spectra of the PVA and dried PVFe-Gel are recorded in the ESI (SI-2†). A broad absorption at 3000–3600 cm−1 corresponds to the O–H stretching vibrations of the hydroxyl groups of PVA. As atactic PVA contains acetate moieties, a >C
O stretching absorption peak of the ester group at 1713 cm−1 was observed. There was a sharp peak at 1090 cm−1 corresponding to the stretching band of the >C–O group of PVA, which overlaps with the Fe–O–C linkage of the gel.42,43
Swelling analysis
Gels were allowed to swell in PBS at 37 °C and weights of the swollen gels were noted at regular intervals. No leaching of the iron ions was observed from the gels during this swelling process. This was also confirmed from the absence of colour around the gels and no detectable iron ions in the medium by elemental analysis. The swelling ratio (Qm) was calculated from the weight of the PBS uptake to the weight of the dried gel. Fig. 1 shows the relationship between the Qm with different amounts of cross-linkers. Qm increased rapidly from 10 to 20 within the first 10 hours, thereafter, swelling slowed significantly.
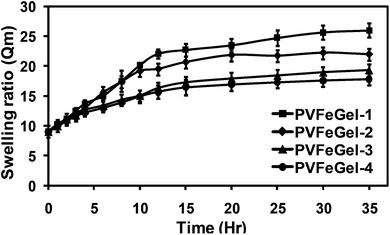 |
| Fig. 1 The swelling ratio of hydrogels for different degrees of crosslinks (i.e. amounts of Fe(III) ions). | |
The volumetric swelling ratio for the PVFeGel-1 showed a maximum up to 26 while for PVFeGel-4 it was 16. These results illustrate that the crosslinking density is directly related to the swelling ratio. Networks with low degrees of crosslinks provide high interchain distances, and are able to retain sufficient amounts of water inside the network. Similarly an increase in the crosslink density brings different chains closure to each other, resulting in lower swelling and a condensed structure. Similarly, the change in the swelling behaviour was investigated in the presence of common cations, such as K+, Na+ and Ca2+ ions. The swelling behaviour was observed up to 5 days at 37 °C and the results are shown in the ESI (SI-3†). From the graph, no change in the swelling behaviour was observed.
Structural evaluation of designed hydrogels
Samples with optimum swelling were lyophilized, sliced and used for SEM analysis. The SEM images of PVFeGel-4 show a highly porous interconnected network structure with an average pore size of 500–800 μm (SI-4A†). Lyophilization allows one to remove the frozen water with the structural frame of the hydrogel with some artifacts, still we can presume that the cavities seen in the SEM indicates the volume occupied by water in the hydrogel network.44–47 Hence we attempted to use an optical microscope to evaluate the hydrogels in the hydrated state. The microscopic image also revealed the porous nature of the hydrogel’s surface (SI-4B†). The hydrogel has a high water retention capacity inside the channels which may allow the diffusion of both small molecules and macromolecules. Furthermore, the high water content of the gel was established as the weight loss during the thermal treatment of the swollen gels using TGA (Fig. 2). From the curve it was found that about 90% of the total weight corresponds to water. This also implies the high water content of the hydrogel matrix.
Mechanical properties of hydrogel
For designing a polymeric biomaterial, the composition and structure of the material relevant to the desired properties should be investigated. Prior to designing a biomaterial, the important properties such as the thermal, physical, mechanical and biological properties need to be thoroughly evaluated. The thermal characteristics during the dynamic processing conditions provide information for stability, shrinkage, expansion and storage. Furthermore, sterilization methods for biomaterials include steam or irradiation before application. So the mechanical properties under different conditions play a vital role during the design of biomaterial scaffolds. DMA is a useful technique for the characterization of biomaterials because it helps to correlate a wide range of material properties. Common techniques for measuring the mechanical properties of swollen hydrogels are static compressive modulus, dynamic mechanical analysis and creep behaviour. All these methods give an idea about the viscoelastic behaviour of the hydrogel.
Stress–strain behaviour of the prepared hydrogels
The mechanical property in static conditions gives an idea about the load bearing capacity during real applications. For this, the uniaxial stress–strain behaviour of coordinated hydrogels in compression mode was carried out and is shown in Fig. 3A. Under an applied stress of 6 Pa, PVFeGel-4 and PVFeGel-1 showed strains of 47% and 62%, respectively (Fig. 3B).
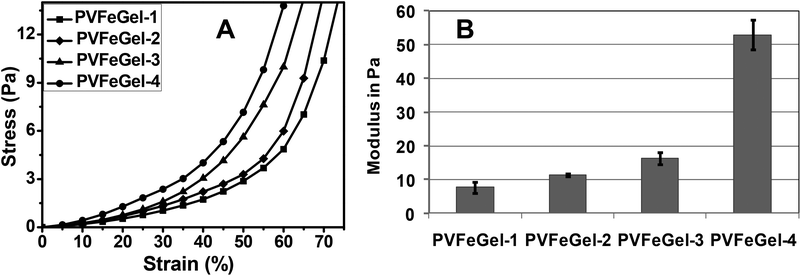 |
| Fig. 3 The stress–strain graph for the hydrogels with different concentrations of crosslinks under uniaxial compression mode (A) and the graph for the compressive modulus of the hydrogels with different concentrations of crosslinks (B). | |
When compressive stress was applied, strain developed locally and dissipated by deforming the hydrogel network. So the less coordinated gel showed a higher strain at constant stress. The modulus of the gel network was calculated up to the elastic limit and was observed around 7.66 ± 1.73 Pa and 52.9 ± 4.4 Pa (Fig. 3B) for PVFeGel-1 and PVFeGel-4, respectively. The physical entanglement of the coordinated network helps to attain its original conformation after releasing the stress, up to the elastic limit. Increasing the crosslinking density leads to the formation of a stiffer network and hence limits the capacity to dissipate the stress imposed during compression. Thus it can be concluded that the gel modulus varies owing to the simple changes in the degree of crosslinks.
Dynamic mechanical analysis
It is important to assess the mechanical properties of hydrogels used for tissue engineering, especially, when they are subjected to external deformations. The dynamic mechanical analyzer correlates the strain induced by the application of constant dynamic stress. The storage modulus (G′) can be correlated with the viscoelastic behaviour of the polymeric hydrogel. The values of G′ at equilibrium for the swollen hydrogel samples are shown in Fig. 4A at 37 °C at different frequencies. It was observed that by increasing the concentration of crosslinking, the G′ of the resulting gel increases.
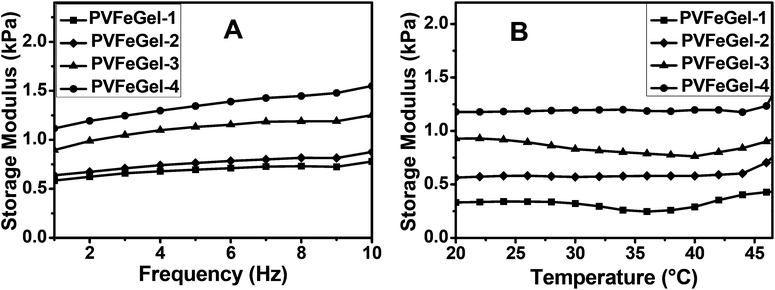 |
| Fig. 4 A graph of the storage modulus vs. frequency of the different hydrogels at biological temperature (37 °C) (A) and a graph for the storage modulus vs. temperature of the hydrogels with different degrees of cross-linkers at constant frequency (B). | |
Furthermore, an increase in G′ can be depicted with a rise in frequency. From the graph, it shows that the G′ for PVFeGel-1 is 0.58 kPa at 1 Hz and 0.78 kPa at 10 Hz, similarly, G′ for PVFeGel-4 is 1.1 kPa at 1 Hz and 1.5 kPa at 10 Hz. An increase in G′ reveals that the elastic response is proportional to the degree of crosslinks and the network structure.
For understanding the material performance, it is important to study the viscoelastic behaviour with change in temperature. For this study, a temperature limit up to 45 °C was employed owing to the dehydration of the gel at higher temperature. Changes in G′ in the temperature range 20–45 °C for all the gels (Fig. 4B) were monitored and only marginal changes in the values of G′ were observed. So the material has no change in elastic response at the same frequency in the temperature range 20–40 °C. For different concentrations of Fe3+ ions, a very low dissipation factor (tan δ ≪ 1) was observed, which indicates viscoelastic nature for the hydrogel.
These studies predicted that swollen hydrogels can maintain viscoelastic behaviour with changes in frequency and has a wide range of working temperatures. Recently, many researchers have paid attention to the materials functions based on mechanical stimulus. These studies give in depth ideas about the structure–property analysis of many advanced applications.48–50 Several hydrogels have been used for nucleus pulposus replacements,14,27 contact lenses,51 targeted drug delivery applications,52,53 cartilage bone32,54 and vocal cord mucosa replacement.32
For all these applications, it is very important to match the viscoelastic behaviour of the synthetic hydrogels with that of the native tissues, when subjected to the dynamic loadings under physiological conditions. The modulus of hydrogels can be controlled by changing the crosslinking density. Furthermore, the mechanical stability revealed from DMA results indicates that hydrogels are stable under ambient conditions.
Creep study
Creep is the fundamental test of a polymeric biomaterial which is directly applicable to product performance. This test gives an idea about the biomaterials recovery after removal of a constant amount of load. Fig. 5A shows the comparative study of PVFeGel-2 to PVFeGel-4, where the applied force was 200 Pa for 5 minutes, then relaxed and monitored for the next 10 minutes.
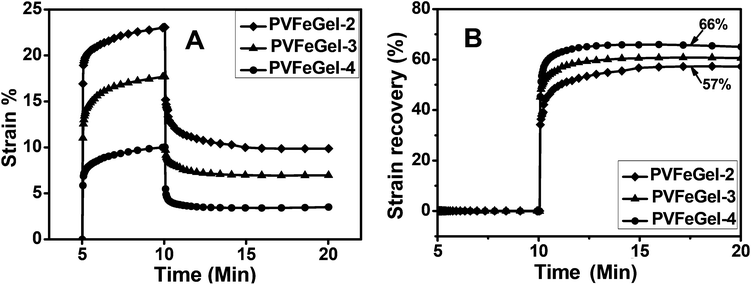 |
| Fig. 5 The creep behaviour of the hydrogels with different concentrations of cross-linkers at biological temperature (37 °C) (A) and a graph for the strain recovery with linear time scale after the release of the applied stress to hydrogels at different concentrations of crosslinks (B). | |
From the graph, it can be seen that there is a quick elastic recovery for all the hydrogels. By applying stress, PVFeGel-2 showed a viscoelastic strain of 23% within 5 minutes, whereas only 10% strain was observed for PVFeGel-4. The high crosslinking density of PVFeGel-4 causes a reduction in the molecular motion and a low strain at the same amount of applied stress. But, for the less crosslinked gel (PVFeGel-2), the movement of chains is easier and a small amount of force can cause a high deformation by retarding elastic response.
Similarly upon releasing the stress, the strain recovery study was carried out for the designed hydrogels (Fig. 5B). Viscoelastic strain recovery was 57% for PVFeGel-2 and 66% for PVFeGel-4. From this study, it can be concluded that there is a quick deformation for gels with a low degree of crosslinks which have less tendency to retract back to their original position. Similarly, a lower amount of viscoelastic strain was developed within 5 minutes for highly crosslinked gels with strong intermolecular bonds (PVFeGel-4), and a high percentage recovery of strain was observed after withdrawing the stress from the hydrogel. This behaviour was in agreement with the high G′ value corresponding to a higher elastic response for PVFeGel-4. Furthermore, the time lag in the development of initial strain and loss in strain after recovery indicated a flow behaviour.55 Hence, the results explain an elastic nature during the application of stress but retarded by viscous resistance. The result obtained from the creep study and storage modulus analysis showed a viscoelastic nature of the hydrogel. As most of the tissues are viscoelastic in nature,33–35 such as nucleus pulposus11,34,35 and other space filling materials,56 a comparative study under different conditions is crucial in evaluating the ultimate property of such materials. Our creep analysis revealed that the hydrogels are viscoelastic in nature and can be tuned by changing the degree of crosslinks.
Several crosslinking methods have been proposed for the development of PVA based hydrogels and their static and dynamic mechanical properties have been investigated.53,54 However, the majority of the crosslinked materials showed a high modulus and low elasticity, which limits their applications in biomedical devices. As compared to the reported method, hydrogels prepared in this study impart low modulus, high viscoelasticity and stability which have many advantages in advanced applications.
Thermal analysis
The purpose of introducing crosslinks in the system is to prevent the flow and to enhance the mechanical integrity of the polymers. However, in between the crosslinks, the molecular segments remain flexible. In particular, the intrinsic chain flexibility and temperature required to induce segmental motion is determined by the nature and amount of crosslinks.
To gain a better understanding on the effect of crosslinks towards the molecular mobility of the designed macromer, thermal analyses of the gels were carried out from room temperature to 250 °C. The glass transition temperature (Tg) was calculated from the second heating cycle for all samples and is shown in Fig. 6. Table 1 shows the summary of the Tg, melting point and melting peak enthalpy of all the hydrogels and pure PVA. The observed high Tg for the PVFeGel-4 at 80.4 °C as compared to pure PVA (72.6 °C) indicates the effect of the high degree of crosslinks. Furthermore, metal coordination with the –OH groups of the PVA backbone (both intra- and interchain) imposes additional constraints on the segmental motion of PVA which led to an increase in Tg. The significant increase in melting point (230 °C) and two-fold increase in melting enthalpy (ΔHm) for PVFeGel-4 in comparison with pure PVA is accountable based on the presence of the high degree of crosslinks.
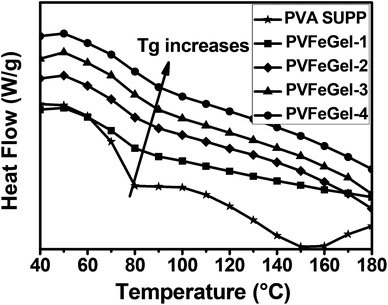 |
| Fig. 6 DSC traces indicating the changes in glass transition temperature at different concentrations of Fe(III) ions in the gel. | |
Table 1 DSC analysis of lyophilized samples of hydrogels
Sample ID |
Glass transition temperature (Tg in °C) |
Melting point (M.P. in °C) |
Melting peak enthalpy (ΔHm in J g−1) |
PVA |
72.6 |
193 |
28.5 |
PVFeGel-1 |
74.2 |
227 |
6.9 |
PVFeGel-2 |
75.3 |
229 |
2.3 |
PVFeGel-3 |
77.1 |
230 |
54.2 |
PVFeGel-4 |
80.4 |
230 |
58.1 |
The melting of a polymer or crosslinked polymer is an endothermic process which absorbs energy for the transition from a semi-crystalline phase (for atactic PVA) to a solid amorphous phase and is directly proportional to the crosslink density. For a PVA gel system, this may be due to the increase in the formation of stable coordination bonds between the Fe(III) ion and hydroxyl groups of PVA. So it can be concluded that by increasing the crosslinks, a higher temperature is needed to increase the polymer chain mobility.
Cytotoxicity analysis
The biological responses of hydrogels are largely controlled by surface functional groups and topography. Hydrogels are hydrophilic polymer networks and can be used as biomaterials with low platelet adsorption, protein adsorption and blood clot formation.57
A highly hydrophilic surface results in low interfacial tension between the hydrogel surface and the surrounding biological fluids.58 Zhang et al. reported that PVA based material showed minimum platelet adherence and no sign of accumulation of pseudopodium.23 Our toxicity investigation of the Fe(III) crosslinked PVA hydrogel was carried out using the IMR-90 cell line. For the cytotoxicity study, Fe(III) crosslinked gels (3 mm diameter and 2 mm in height) were placed inside a 24-well culture plate and seeded with IMR-90 cells. The plates were incubated and cell growth inside the well was monitored. After 5 days, the cells were proliferating smoothly with no observable toxicity effect by the hydrogels. The cell morphology was found to be normal (Fig. 7A) indicating that the hydrogel has no cytotoxic effect towards the surrounding media. Furthermore, cell viability data revealed that there was almost no enhancement or decrease in cell growth in the well with hydrogels as compared to the control (Fig. 8) after 5 days of incubation. These results suggest that the hydrogels are free from any toxic leachable materials.
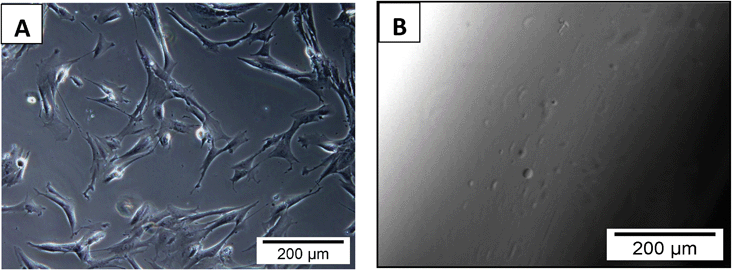 |
| Fig. 7 Optical micrographs showing cell proliferation of IMR-90 cells in the cell culture well around the hydrogels (A) and absence of cell growth on the surface of the hydrogel (B) after 5 days of co-culture. | |
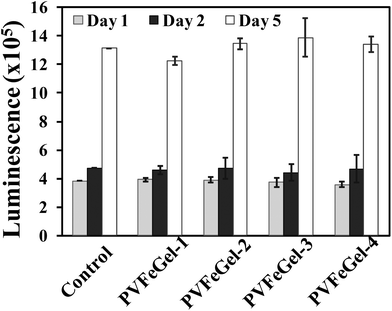 |
| Fig. 8 Cell viability test with IMR-90 on crosslinked hydrogels with up to 5 days of analysis. The data represents the mean and standard deviations for four samples. The control experiment was normalised as same cell line grown with different time periods. | |
Cell adhesion study
Similarly, for the cell adhesion study, cells were seeded on the surface of the designed hydrogels. Typically, PVA gels with different degrees of crosslinking were used for the cell adhesion studies. After seeding cells on the gel surface, plates were incubated at 37 °C for 5 days. No cell growth on the surface of the crosslinked gels (Fig. 7B) was observed. From the above study, it was concluded that hydrogels are not cytotoxic in nature and do not allow cell adhesion on their surfaces owing to the hydrophilic nature of PVA.
Conclusions
The present investigation focused on the investigation of the structure–property relationship of the Fe(III) crosslinked PVA hydrogel system. Simple changes in the formulation of the hydrogels led to significant differences in the final properties of the material. No leaching of the Fe3+ ions was observed during our repeated swelling and drying cycles. From the swelling studies, it was observed that the Qm decreased with an increase in crosslinking density. Thermal analysis revealed an increase in Tg, melting point and melting enthalpy with increase in concentration of Fe3+ ions. Mechanical studies highlighted the viscoelastic nature of the gel. Furthermore, viscoelastic response and relaxation behaviour were greatly influenced by the amount of crosslinks. The temperature dependent dynamic mechanical study showed no significant change in the modulus up to 45 °C. The as prepared hydrogels were nontoxic towards IMR-90 fibroblast cells, and no significant cell attachment was observed on the hydrogel surface. Such hydrogels may find practical applications which need long term stability with high viscoelastic behaviour. Extensive biological evaluation needs to be done to use these materials for real applications.
Acknowledgements
The authors acknowledge the support from the Department of Chemistry, National University of Singapore (NUS). NM thanks NUS for the graduate fellowship. YT acknowledges a PhD scholarship from the NUS-graduate school.
References
- J. Kopeček and J. Yang, Polym. Int., 2007, 56, 1078–1098 CrossRef
.
- R. Langer, Science, 1990, 249(4976), 1527–1533 CAS
.
- A. S. Sawhney, C. P. Pathak and J. A. Hubbell, Macromolecules, 1993, 26, 581–587 CrossRef CAS
.
- J. L. West and J. A. Hubbell, React. Funct. Polym., 1995, 25(2/3), 139–147 CAS
.
- S. H. Hyon, W. I. Cha, Y. Ikada, M. Kita, Y. Ogura and Y. Honda, J. Biomater. Sci., Polym. Ed., 1994, 5, 397–406 CrossRef CAS
.
- K. Ariga, Q. Ji, G. J. Richards and J. P. Hill, Soft Mater., 2012, 10, 387–412 CrossRef CAS
.
- S. Matsumoto, S. Yamaguchi, S. Ueno, H. Komatsu, M. Ikeda, K. Ishizuka, Y. Iko, K. V. Tabata, H. Aoki, S. Ito, H. Noji and I. Hamachi, Chem.–Eur. J., 2008, 14, 3977–3986 CrossRef CAS
.
- W. E. Hennink and C. F. van Nostrum, Adv. Drug Delivery Rev., 2002, 54, 13–36 CrossRef CAS
.
-
M. S. Shoichet and J. A. Hubbell, Polymers for Tissue Engineering, VSP BV, The Netherlands, 1998, 235–254 Search PubMed
.
- E. De Carlo, S. Baiguera, M. T. Conconi, S. Vigolo, C. Grandi, S. Lora, C. Martini, P. Maffei, G. Tamagno, R. Vettor, N. Sicolo and P. P. Parnigotto, Int. J. Mol. Med., 2010, 25, 195–202 CAS
.
- N. Mahanta and S. Valiyaveettil, RSC Adv., 2012, 2, 11389–11396 RSC
.
- L. E. Millon, H. Mohammadi and W. K. Wan, J. Biomed. Mater. Res., Part B, 2006, 79B, 305–311 CrossRef CAS
.
- C. R. Nuttelman, D. J. Mortisen, S. M. Henry and K. S. Anseth, J. Biomed. Mater. Res., 2001, 57, 217–223 CrossRef CAS
.
- N. Mahanta, Y. Teow and S. Valiyaveettil, J. Nanosci. Nanotechnol., 2012, 12, 6156–6162 CrossRef CAS
.
- P. E. Le Renard, O. Jordan, A. Faes, A. Petri-Fink, H. Hofmann, D. Rufenacht, F. Bosman, F. Buchegger and E. Doelker, Biomaterials, 2010, 31, 691–705 CrossRef CAS
.
- P. Basak and B. Adhikari, J. Mater. Sci.: Mater. Med., 2009, 20, 137–146 CrossRef
.
- T. Koyano, N. Koshizaki, H. Umehara, M. Nagura and N. Minoura, Polymer, 2000, 41, 4461–4465 CrossRef CAS
.
- W. Paul and C. P. Sharma, J. Biomater. Sci., Polym. Ed., 1997, 8, 755–764 CrossRef CAS
.
- J. Wang, C. Gao, Y. Zhang and Y. Z. Wan, Mater. Sci. Eng., C, 2010, 30, 214–218 CrossRef CAS
.
- K. C. Chu and B. K. Rutt, Magn. Reson. Med., 1997, 37, 314–319 CrossRef CAS
.
- M. Kobayashi and M. Oka, J. Biomater. Sci., Polym. Ed., 2004, 15, 741–751 CrossRef CAS
.
- Y. S. Pan and D. S. Xiong, Wear, 2009, 266, 699–703 CrossRef CAS
.
- T. Jiang, G. X. Wang, J. H. Qiu, L. L. Luo and G. Q. Zhang, J. Med. Biol. Eng., 2009, 29, 102–107 Search PubMed
.
-
A. Atala, D. J. Mooney, J. P. Vacanti and R. Langer, Synthetic biodegradable polymer scaffolds, Birkhauser, Boston, 1997 Search PubMed
.
- C. M. Hassan and N. A. Peppas, Adv. Polym. Sci., 2000, 153, 37–65 CrossRef CAS
.
- S. Kurihara, S. Sakamaki, S. Mogi, T. Ogata and T. Nonaka, Polymer, 1996, 37(7), 1123–1128 CrossRef CAS
.
- N. Mahanta, W. Y. Leong and S. Valiyaveettil, J. Mater. Chem., 2012, 22, 1985–1993 RSC
.
- P. Martens and K. S. Anseth, Polymer, 2000, 41, 7715–7722 CrossRef CAS
.
- P. Martens, T. Holland and K. S. Anseth, Polymer, 2002, 43, 6093–6100 CrossRef CAS
.
- F. Cavalieri, F. Miano, P. D’Antona and G. Paradossi, Biomacromolecules, 2004, 5, 2439–2446 CrossRef CAS
.
- A. Muhlebach, B. Muller, C. Pharisa, M. Hofmann, B. Seiferling and D. Guerry, J. Polym. Sci., Part A: Polym. Chem., 1997, 35, 3603–3611 CrossRef
.
- S. W. Lee, Y. I. Son, C. H. Kim, J. Y. Lee, S. C. Kim and Y. W. Koh, Laryngoscope, 2007, 117, 1871–1875 CrossRef CAS
.
- A. Muliana and K. R. Rajagopal, Int. J. Solids Struct., 2012, 49, 989–1000 CrossRef CAS
.
- A. Gloria, A. Borzacchiello, F. Causa and L. Ambrosio, J. Biomater. Appl., 2012, 26, 745–759 CrossRef CAS
.
- C. Eyholzer, A. B. de Couraca, F. Duc, P. E. Bourban, P. Tingaut, T. Zirnmermann, J. A. E. Manson and K. Oksman, Biomacromolecules, 2011, 12, 1419–1427 CrossRef CAS
.
-
C. A. Finch, Polyvinyl alcohol: properties and applications, Wiley, London, New York, 1973 Search PubMed
.
- N. K. Mongia, K. S. Anseth and N. A. Peppas, J. Biomater. Sci., Polym. Ed., 1996, 7(12), 1055–1066 CrossRef CAS
.
- S. H. Imam, L. J. Mao, L. Chen and R. V. Greene, Starch/Staerke, 1999, 51(6), 225 CrossRef CAS
.
-
N. A. Peppas, Hydrogels in medicine and pharmacy, CRC Press Inc., Boca Raton, Florida, 1986, vol. 1 Search PubMed
.
- R. B. Hernandez, A. P. Franc, O. R. Yola, A. Lopez-Delgado, J. Felcman, M. A. L. Recio and A. L. R. Merce, J. Mol. Struct., 2008, 877, 89–99 CrossRef CAS
.
- H. Yokoi, Y. Mori and Y. Fujise, Bull. Chem. Soc. Jpn., 1995, 68, 2061–2065 CrossRef CAS
.
- M. V. Nesterova, S. A. Walton and J. Webb, J. Inorg. Biochem., 2000, 79, 109–118 CrossRef CAS
.
- N. Mahanta and S. Valiyaveettil, RSC Adv., 2013, 3, 2776–2783 RSC
.
- S. Petrusic, P. Jovancic, M. Lewandowski, S. Giraud, B. Bugarski, J. Djonlagic and V. Koncar, J. Polym. Res., 2012, 19, 9979 CrossRef
.
- Y. J. Huang, M. Z. Liu, J. C. Chen, C. M. Gao and Q. Y. Gong, Eur. Polym. J., 2012, 48, 1734–1744 CrossRef CAS
.
- G. H. He, Z. Wang, H. Zheng, Y. H. Yin, X. Xiong and R. Y. Lin, Carbohydr. Polym., 2012, 90, 1614–1619 CrossRef CAS
.
- C. Chang, N. Peng, M. He, Y. Teramoto, Y. Nishio and L. Zhang, Carbohydr. Polym., 2013, 91, 7–13 CrossRef CAS
.
- K. Ariga, T. Mori and J. P. Hill, Adv. Mater., 2012, 24, 158–176 CrossRef CAS
.
- T. Aida, E. W. Meijer and S. I. Stupp, Science, 2012, 335, 813–817 CrossRef CAS
.
- T. Jiang, G. X. Wang, J. H. Qia, L. L. Luo and G. Q. Zhang, Chem. Soc. Rev., 2012, 41, 1111–1129 RSC
.
- N. Mahanta and S. Valiyaveettil, Nanoscale, 2011, 3, 4625–4631 RSC
.
- S. Y. Lee, B. P. Pereira, N. Yusof, L. Selvaratnam, Z. Yu, A. A. Abbas and T. Kamarul, Acta Biomater., 2009, 5, 1919–1925 CrossRef CAS
.
- J. K. Wu, X. L. Gong, Y. C. Fan and H. S. Xia, Soft Matter, 2011, 7, 6205–6212 RSC
.
- L. E. Millon, D. T. Padavan, A. M. Hamilton, D. R. Boughner and W. K. Wan, J. Biomed. Mater. Res., Part B, 2012, 100B, 1–10 CrossRef CAS
.
- P. Soltani, M. M. Taherian and A. Farshidianfar, J. Phys. D: Appl. Phys., 2010, 43, 425401 CrossRef
.
- Food and Drug Administration, Blood Serum Chemistry - Normal Values, Appendix C, 2012, 428–429; http://www.fda.gov/downloads/ICECI/Inspections/IOM/UCM135835.pdf Search PubMed.
- Y. L. Li, K. G. Neoh and E. T. Kang, Polymer, 2004, 45, 8779–8789 CrossRef CAS
.
- H. Jiang, G. Campbell, D. Boughner, W. K. Wan and M. Quantz, Med. Eng. Phys., 2004, 26, 269–277 CrossRef
.
Footnote |
† Electronic supplementary information (ESI) available: camera images of the clear solution of PVA and the hydrogel formed in the presence of Fe(III) ions (SI-1). Attenuated total reflectance-infrared spectra of the lyophilized sample of (a) PVA and (b) Fe3+ crosslinked PVA (SI-2). Swelling analysis of hydrogels in the presence of common cations such as Na+, K+ and Ca2+ at different concentrations (SI-3). Scanning electron micrographs of the freeze dried lyophilized sample of PVFeGel-4 (A) and the optical microscopic image of the water swollen hydrogel of PVFeGel-4 (B) (SI-4). See DOI: 10.1039/c3bm00167a |
|
This journal is © The Royal Society of Chemistry 2013 |
Click here to see how this site uses Cookies. View our privacy policy here.