DOI:
10.1039/C2PY20692G
(Paper)
Polym. Chem., 2013,
4, 68-75
Factors influencing the growth and topography of nanoscale films fabricated by ROMP-mediated continuous assembly of polymers†
Received
28th August 2012
, Accepted 10th September 2012
First published on 13th September 2012
Abstract
The continuous assembly of polymers (CAP) mediated via ring-opening metathesis polymerization (ROMP) is demonstrated as a simple and versatile method to fabricate tailored nanostructured thin films. The film thickness and topography were highly dependent upon the variation of different factors that influence the ROMP reaction and mechanism of the assembly process. Herein, we present a detailed investigation of the influence of various parameters on the rate of film formation, the film thickness and the film topography. Whereas the macrocross-linker concentration and molecular weight determined the final film thickness and surface coverage, the initiator concentration and ROMP catalyst activity were found to have a negligible effect on the film properties. Importantly, the minimum amount of polymerizable moieties required in the macrocross-linker to obtain fine control over film thickness and high surface coverage was found to be 7 mol%. The addition of excess ligand (≤100 mM) for the catalyst increased the catalyst lifetime leading to thicker films, although further increases (>100 mM) were found to retard the metathesis reaction. These findings provide valuable insights into the CAPROMP process and will contribute toward developing the next generation of CAP ultrathin films for advanced applications.
Introduction
Over the last few decades, engineered soft nanostructures, such as polymeric films on surfaces, have enabled the exploration and exploitation of nanoscale phenomena.1,2 Such advancements have been aided by the development of conventional techniques, such as polymer grafting-to and -from, which allow the generation of well-controlled and structured films on surfaces.3–5 Although these techniques allow the generation of well-defined films, both grafting techniques have limitations. In grafting-to, diffusion or steric hindrance is a major issue and generally leads to very thin (<10 nm), low rigidity films with poor stability, particularly for high molecular weight polymers.4 In comparison, the grafting-from approach – that mainly utilizes controlled polymerization techniques to produce tailored polymeric brushes on surfaces – suffers from the limitation to generate films derived from complex polymer architectures (e.g., stars and dendrimers) or compositionally unique molecules (e.g., natural biomolecules).3 A significant breakthrough in nanoscale assembly that resolves the limitations associated with conventional grafting techniques is layer-by-layer (LbL) assembly.6–8 Although LbL assembly allows the preparation of films with controlled thickness and nanometer resolution, the mechanism of film formation relies on the sequential deposition of complementary functionalized polymers, which can be time and labor intensive.7 In addition, several groups have employed distillation precipitation polymerization (DPP), which relies on the addition of oligomeric radicals (generated in situ from vinyl monomers and cross-linkers) to vinyl groups present on a substrate, followed by polymerization at the surface.9,10 Although the DPP approach generates cross-linked films in a single-step, it is difficult to control the density, thickness and hence the permeability of the films, and is limited in its selection of monomers and cross-linkers. Furthermore, the generation of free radicals in solution may lead to the formation of additional insoluble polymeric particles that could be difficult to remove.9,10 Messersmith and co-workers introduced a technique that is able to generate polydopamine films on a wide range of substrates using a single-dip coating procedure, which allows control over film thickness via immersion time.11 However, this approach is only applicable to the generation of polydopamine (or other catechol derivative) films and the process is not surface-confined, as there is also a tendency for polymerization to occur in solution.11 Another bioassembly technique developed by Mertz et al. involves the single-step assembly of a wide variety of biopolymeric architectures (hollow capsules and replicated spheres or fibers) via non-covalent interactions (hydrogen and halogen bonding) with bromoisobutyramide (BrIBAM)-modified substrates.12 This approach produces free-standing biopolymeric films, which are difficult to achieve using other methods.12
Recently, we reported a novel film fabrication strategy, referred to as continuous assembly of polymers (CAP), mediated by ring-opening metathesis polymerization (ROMP), which involves the one-step assembly (and polymerization) of macrocross-linkers – (bio)macromolecules functionalized with polymerizable moieties – to afford surface-confined, cross-linked, ultrathin films with tailored properties (Scheme 1). The CAP approach offers the possibility of combining the benefits of polymer grafting methods, while simultaneously overcoming their limitations. In addition, the CAP approach allows continuous cross-linked film formation similar to the DPP and dopamine systems, while maintaining surface confinement, and also eliminates the need for multiple washing and deposition steps.13–15 Herein, we report a detailed investigation of various factors that affect film formation and properties in the ROMP-mediated continuous assembly of polymers (CAPROMP) process (Scheme 1), including the influence of the catalyst (type, concentration and presence of additives) and the macrocross-linker (molecular weight, concentration and amount of polymerizable norbornene groups). The rate of film growth and surface roughness/homogeneity of macrocross-linker P1 films are characterized and compared by ellipsometry and atomic force microscopy (AFM).
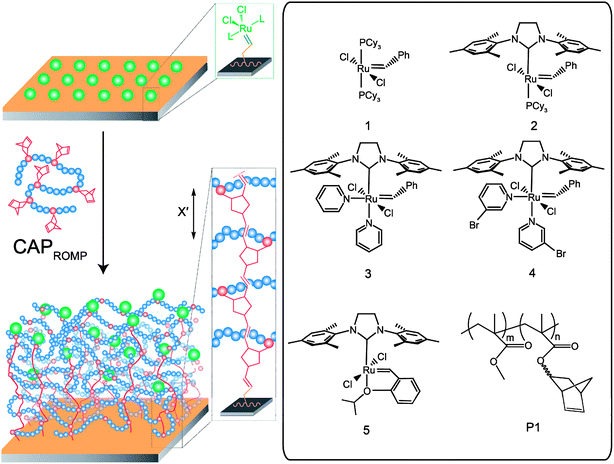 |
| Scheme 1 CAPROMP approach using macrocross-linker P1 and initiated by surface-bound metathesis catalysts 1–5. The polymer chain spacing is relative to the norbornene (X′) repeating unit size. | |
Experimental section
Materials
Allyl bromide (99%), calcium hydride (CaH), N,N′-dicyclohexylcarbodiimide (DCC, 99%), 4-(dimethylamino)pyridine (DMAP, ≥ 99%), ethyl vinyl ether (EVE, 99%), 5-norbornen-2-ol (mixture of endo and exo, 99%), methacryloyl chloride (97%) and poly(ethylene imine) (PEI) (Mw ∼25.0 kDa) were obtained from Aldrich and used without further purification. Methyl methacrylate (MMA, 99%) was obtained from Aldrich, passed over plugs of inhibitor remover (Aldrich) twice to remove any inhibitors present and stored below 4 °C prior to use. Metathesis catalyst (IMesH2)(Cl)2(C5H5N)2Ru
CHPh (catalyst 3) was prepared from the 2nd generation Grubbs catalyst (Aldrich), as described in the literature.16 Pyridine was obtained from Scharlau and used without further purification. 2,2′-Azobis(2-methylpropionitrile) (AIBN, 98%) was obtained from Acros and used without further purification. Magnesium sulphate (MgSO4, anhydrous), n-hexane, toluene, isopropanol and ethanol were obtained from Merck and used without further purification. Diethyl ether (DEE) and sodium hydroxide (NaOH) were obtained from Chem-Supply and used without further purification. Anhydrous, deoxygenated dichloromethane (DCM) and tetrahydrofuran (THF) were obtained by distillation under argon from CaH and sodium benzophenone ketyl, respectively. Deuterated chloroform (CDCl3), methanol (CD3OD) and dimethylsulfoxide (d6-DMSO) were obtained from Cambridge Isotope Laboratories. High-purity water with a resistivity greater than 18 MΩ cm was obtained from an in-line Millipore RiOs/Origin water purification system.
Silicon wafers (MMRC Pty. Ltd, Melbourne, Australia) were cut to approximately 1 × 1 cm slides and cleaned with Piranha solution (sulphuric acid
:
hydrogen peroxide = 7
:
3). Caution!Piranha solution is highly corrosive and extreme care should be taken during preparation and use. The slides were then sonicated in isopropanol:water (1
:
1) solution for 20 min and finally heated to 60 °C for 20 min in RCA solution (water:ammonia:hydrogen peroxide = 5
:
1
:
1). The slides were washed thoroughly with Milli Q water between each step.
Measurements
Monomer conversion was determined by GC analysis on a Shimadzu GC-17A gas chromatograph equipped with a DB-5 capillary column (Phenomenex, solid phase 5% phenylsiloxane and 95% dimethylpolysiloxane; 30 m × 0.25 mm × 0.25 μm) and coupled to a GC-MS-QP5000 electron ionization mass spectrometer. Samples taken from reaction mixtures were diluted with an appropriate amount of THF and injected directly into the GC.
Polymer molecular weight characterization was carried out via GPC using a Shimadzu liquid chromatography system coupled to a Wyatt DAWN EOS MALLS detector (658 nm, 30 mW) and Wyatt OPTILAB DSP interferometric refractometer (658 nm), and using three Phenomenex Phenogel columns in series (500, 104 and 106 Å porosity; 5 μm bead size) operating at 30 °C. THF was used as the eluent at a flow rate of 1 mL min−1. Aliquots (0.5 mL) from each reaction mixture were diluted with an appropriate amount of THF and passed through a 0.45 μm filter and injected into the GPC for analysis. Astra software (Wyatt Technology Corp.) was used to determine the molecular weight characteristics using known dn/dc values.17
1H and 13C NMR measurements were conducted on a Varian Unity 400 MHz spectrometer at 400 and 100 MHz, respectively, using the deuterated solvent as reference and a sample concentration of ca. 20 mg mL−1.
Ellipsometry measurements of the CAP coated films were performed on a UVISEL spectroscopic ellipsometer from Jobin Yvon. Spectroscopic data were acquired between 400–800 nm with a 2 nm increment, and thicknesses were extracted with the integrated software by fitting with a classical wavelength dispersion model.18
Atomic force microscopy (AFM) images of air-dried CAPROMP films on silicon wafers were acquired with an MFP-3D Asylum Research instrument. Typical scans were conducted in AC mode with ultrasharp SiN gold-coated cantilevers (MikroMasch, Bulgaria). Image processing and surface roughness analysis were performed using the Nanoscope and Igor Pro software programs, respectively. CAP film thicknesses were estimated by film scratching (mechanical removal) and by tracing a profile along the film and the scratched zone. The thickness measurements reported represent mean values over 3 different analysis sites per substrates and physical analysis of scratched films by AFM showed good agreement with ellipsometry data.
Procedures
Synthesis of bicyclo[2.2.1]hept-5-en-2-yl methacrylate (BHEMA).
This compound was prepared according to a previously published procedure.131H NMR (400 MHz, CDCl3, TMS) (endo) δH 6.33 (dd, 1H,
CH), 5.97–6.00 (m, 2H,
CH + CHH), 5.49 (s, 1H, CHH), 5.31–5.34 (m, 1H, CHO), 3.17 (br s, 1H, CH), 2.85 (br s, 1H, CH), 2.13–2.19 (m, 1H, CHH), 1.88 (s, 3H, CH3), 1.43–1.51 (m, 1H, CHH), 1.34 (d, 1H, CHH), 0.96 (dt, 1H, CHH) ppm; (exo) δH 6.25 (dd, 1H,
CH), 6.08 (s, 1H, CHH), 5.97–6.00 (m, 1H,
CH), 5.53 (s, 1H, CHH), 4.72–4.73 (m, 1H, CHO), 2.92 (br s, 1H, CH), 2.85 (br s, 1H, CH), 2.43 (ddd, 1H, CHH), 1.94 (s, 3H, CH3), 1.67–1.74 (m, 1H, CHH), 1.58–1.63 (m, 1H, CHH), 1.43–1.51 (m, 1H, CHH) ppm; 13C NMR (100 MHz, CDCl3, TMS) (endo) δC 167.8 (CO), 138.6 (
CH2), 136.8 (
C(CH3)), 131.7 (
CH), 125.2 (
CH2), 75.5 (CHO), 47.8 (CH2), 46.0 (CH), 42.4 (CH), 34.8 (CH2), 18.4 (CH3) ppm; (exo) δC 167.8 (CO), 141.3 (
CH), 137.0 (
C(CH3)), 132.8 (
CH), 125.2 (
CH2), 75.6 (CHO), 47.5 (CH2), 46.5 (CH), 40.8 (CH), 34.9 (CH2), 18.5 (CH3) ppm. Ratio of exo:endo (%) = 27
:
73.
Synthesis of norbornene functionalized PMMA macrocross-linker P1 (poly(methyl methacrylate-ran-(bicyclo[2.2.1]hept-5-en-2-yl methacrylate))).
This compound was prepared according to a previously published procedure.13 In a typical experiment, MMA (4.06 mL, 0.04 mol), BHEMA (0.75 g, 0.004 mol) and AIBN (0.08 g, 0.0005 mol) were dissolved in toluene (12.4 mL). The reaction mixture was deoxygenated via three freeze–pump–thaw cycles and then submerged in a 100 °C oil bath for 2 h. The reaction mixture was then diluted with THF (12.5 mL) and precipitated into cold methanol (75 mL) to yield polymer P1 as a white solid, 3.02 g (65%); GPC-MALLS (THF): Mw = 15.9 kDa, Mw/Mn = 1.77; 1H NMR (400 MHz, CDCl3, TMS) δH 6.26–6.36 (m,
CH), 5.99 (br s,
CH), 5.27 (br s, CHO), 4.57 (br s, CHO), 3.60 (br s, CH3O), 3.10 (br s, CH), 2.88 (br s, CH), 2.49 (br s, CHH), 1.81–1.90 (m, CH2), 1.43 (br, CH3), 1.24–1.27 (m, CH3), 1.02 (br s, CH3), 0.84 (br s, CH3) ppm. The pendent norbornene functionality was 7 mol%, as determined by 1H NMR spectroscopic analysis. In order to obtain macrocross-linkers with different mol% of pendent norbornene groups the amount of BHEMA was varied between 0.002 mol (3 mol% norbornene), 0.008 mol (15 mol% norbornene) and 0.036 mol (34 mol% norbornene), while the concentration of other reagents was kept constant. To obtain different molecular weight macrocross-linkers, identical amounts of monomers were used, however, the amount of AIBN was varied from 0.1 mmol (72.9 kDa) to 2 mmol (6.2 kDa). All reactions were conducted at 100 °C for 2 h with the exception of the macrocross-linker with a molecular weight of 72.9 kDa, for which the reaction time was increased to 18 h.
Synthesis of hyperbranched poly(N-allyl ethylene imine) (allyl-PEI).
This compound was prepared according to a previously published procedure.131H NMR (400 MHz, CD3OD) δH 5.85 (br s, CH2
CHCH2N), 5.17–5.22 (m, CH2
CHCH2N), 3.11–3.20 (m, CH2
CHCH2N), 2.57 (br s, N(CH2)2N) ppm. The allyl functionality was 30 mol%, as determined by 1H NMR spectroscopic analysis.
Assembly of CAPROMP films on planar substrates.
All planar substrate manipulations were conducted in individual oven-dried 7 mL vials under argon. Si wafers (ca. 1 × 1 cm) functionalized with catalyst 1–5 (details of this functionalization are provided elsewhere13) were placed in vials followed by the addition of 1 mL of 1 mM CAP-active macrocross-linker P1 in anhydrous and degassed dichloromethane (DCM). After standing at room temperature for a predetermined time the polymer-coated wafers were removed (different thickness films were obtained by variation of the exposure time), washed with DCM (3 × 20 mL) and then exposed to 2% v/v ethyl vinyl ether (EVE) in DCM (5 mL) as a capping solution to remove the Ru catalyst from the surface of the films for 12 h, before finally being washed and dried in vacuo prior to analysis.
Results and discussion
The CAP process involves the exposure of an initiator-functionalized substrate to a solution containing a macrocross-linker, resulting in the polymerization of the macrocross-linker from the surface and the formation of cross-linked nanoscale films. In theory, CAP film formation could be influenced by a number of parameters, including the concentration and composition of the macrocross-linkers and also factors that have an effect on the controlled polymerization technique that is used. Therefore, this study examines the effect of various catalyst and macrocross-linker variables on film formation. The ‘standard’ CAPROMP conditions were similar to our earlier study,13 which employed poly(methyl methacrylate) (PMMA) P1 as the macrocross-linker with a mass-average molecular weight (Mw) of 15.9 kDa and 7 mol% pendent norbornene functionalities at a concentration of 1 mM. The substrates (silicon wafers) were initially functionalized with an allyl-modified poly(ethylene imine) (allyl-PEI) followed by cross-metathesis using a 1 mM solution of the modified 2nd generation Grubbs catalyst 3 (Scheme 1). CAPROMP was performed without the addition of any additives.
In the following section, the influence of various catalyst parameters on the CAPROMP reaction is presented. This includes the choice of ROMP catalyst, the catalyst concentration and the addition of excess labile ligand in solution.
ROMP catalyst type.
Ruthenium carbene complexes (termed Grubbs catalysts) are highly versatile catalysts due to their low oxophilic characteristic, which makes them stable toward polar functional groups. They are also highly tolerant toward moisture, air and acidic conditions. This enables ROMP to be effective in a variety of solvents, including aqueous or protic media.19 More importantly, these ruthenium-based catalysts are commercially available. Therefore in this study, a variety of ruthenium catalysts were investigated to assess their efficiency in the CAPROMP approach, including the commercially available 1st generation ([(PCy3)2(Cl)2Ru
CHPh)]) and 2nd generation ([(H2IMes)(PCy3)(Cl)2Ru
CHPh)]) Grubbs catalysts (1 and 2, respectively), the modified 2nd generation Grubbs catalyst with bispyridine ([(H2IMes)(py)2(Cl)2Ru
CHPh)]) (3) or bis(3-bromopyridine) ([(H2IMes)(3-Br-py)2(Cl)2Ru
CHPh)]) (4) ligands and the 2nd generation Hoveyda–Grubbs catalyst ([(H2IMes)(Cl)2Ru
CH(o-iPrOC6H4)]) (5) (Scheme 1).
It has been reported previously that catalyst 1 has a higher initiation rate compared to catalyst 2.20 However, the rate of metathesis facilitated by catalyst 2 is two orders of magnitude greater than catalyst 1 due to strong electron donation of the N-heterocyclic carbene (NHC) ligand, which stabilizes the active species.21 In comparison, the modified versions of catalyst 2 (i.e., catalysts 3 and 4) with pyridyl-ligands exhibit increased initiation rates by several orders of magnitude, while showing high reactivity for metathesis reactions.22–24 The Hoveyda–Grubbs catalyst 5 has approximately the same initiation rate as catalyst 2, however, the rebinding of the styrenyl ether ligand to the metal centre is less efficient than the phosphine ligand.25–28 Therefore, for certain reactions, especially those that involve electron-withdrawing carbenes, catalyst 5 facilitates more productive metathesis.26
Fig. 1a illustrates the film thickness profiles for the CAPROMP reactions performed using substrates functionalized with catalysts 1–5, as determined by ellipsometry. All of the substrates were functionalized using 1 mM catalyst solutions and the CAP process was conducted using the previously described standard conditions (vide supra). After 25 h, it was observed that catalyst 1 gave the lowest thicknesses because the metathesis rate is the lowest among the catalysts investigated. Additionally, previous studies have shown that in ROMP mediated by this type of catalyst, exo-isomers of norbornene derivatives are much more reactive than the endo-isomers.29–31 Hence, lower film thicknesses may also be caused by the conformation of the norbornene groups, which were a mixture of endo- and exo- forms. However, the deviation of this final thickness (ca. 100 nm) from the maximum thickness obtained by other catalysts was <20%. Although the variation in the final film thicknesses or the rate of formation is not significantly different for any of the catalysts investigated, AFM images of the final films (Fig. 1b) revealed that only catalyst 3 provided continuous surface coverage, while the resulting films formed from other catalysts were observed to be non-continuous (refer to Fig. S1 and S2 in ESI† for 3D height mode view and scratch analysis, respectively). The poor surface coverage obtained using catalyst 1 most likely results from instability of the active form of the catalyst, while the slow initiation rate associated with catalyst 2 produces an uneven growth rate of the CAP film (Fig. 1b). The film topography and coverage obtained using the bromopyridine-ligated catalyst 4, which exhibits a very rapid initiation rate, is comparable to the film obtained using the Hoveyda–Grubbs catalyst 5. This is expected as a result of the high activity of catalyst 4, which resembles that of catalyst 5 in surface polymerizations, since 5 is always in its active form for metathesis due to the permanent loss of the i-PrOAr ligand after reaction of 5 with the surface allyl groups. The highly active nature of these metathesis catalysts may cause these systems to have uncontrolled growth from the surface and be more susceptible to catalyst decomposition via the coupling reaction between two Ru alkylidenes, which explains the incomplete surface coverage observed (Fig. 1b).32,33 As validated by the experimental data, it can be generalized that the catalyst type affects CAPROMP performance, particularly in terms of film topography and homogeneity. Catalyst 3 was found to perform most efficiently in this system to produce films with high surface coverage within the range of analysis and hence was the catalyst of choice in subsequent studies.
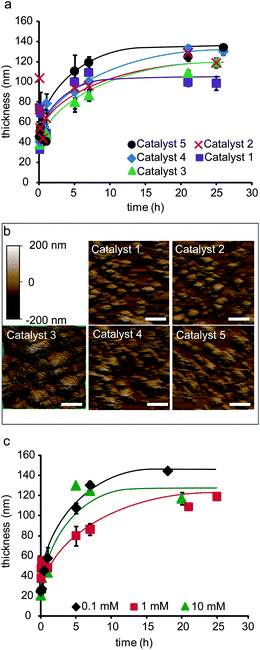 |
| Fig. 1 Effect of ROMP catalyst parameters on CAPROMP: (a) film thickness as a function of time of CAP films prepared using different ROMP catalysts, as determined by ellipsometry; (b) height-mode AFM images (scale bar = 1 μm) of P1 films prepared using different ROMP catalysts (after 25 h); and (c) film thickness as a function of time of CAP films prepared from substrates functionalized using different concentrations of catalyst 3, as determined by ellipsometry. Error bars: ±standard deviation (n = 3). Lines are to guide the eye. | |
Catalyst concentration.
For an efficient CAPROMP reaction to take place, it is important to ensure that the catalyst reacts with as many available allyl groups of the allyl-PEI deposited on the surface of the substrate as possible, such that the number of initiating sites is maximized to allow for efficient film growth. Hence, the rate of film growth and final surface coverage of the films would serve as a reliable indicator to determine if the optimum catalyst concentration has been employed to functionalize the substrate with initiating groups. Fig. 1c shows the rate of film growth for substrates prepared with different catalyst concentrations (10, 1, and 0.1 mM). At all catalyst concentrations used, the kinetic profiles were similar, with only slight differences in the assembly rate and final film thickness (120 to 140 nm), as measured by ellipsometry. The obtained film thicknesses were also validated by AFM scratch analysis, which provided values on average, within the range of those obtained by ellipsometry. The film topography of the CAP films, as examined by AFM, revealed the formation of films with a similar surface roughness over a 5 μm2 area, which is approximately 60 nm (ESI, Fig. S2†). This indicates that the accessible allyl groups on the surface are adequately saturated with catalyst even at a concentration of 0.1 mM. It is likely that variation of the density of surface allyl groups would influence the number of initiators deposited on the surface and, as a result, affect the resultant film properties. However, quantification of the surface density of allyl groups is challenging and is a subject of ongoing studies.
Excess labile ligand.
Pyridine is the labile ligand of catalyst 3 that dissociates and rebinds readily with the Ru metal centre. Upon dissociation, the ruthenium carbene becomes metathesis-active, but upon rebinding, the catalyst returns to its dormant state. Bielawski and Grubbs demonstrated that the addition of excess labile ligand in ROMP affected this equilibrium rate (favoring formation of the dormant species) and consequently limited the propagation rate of the polymerization.34 Hence, addition of excess pyridine (with respect to catalyst 3) to the CAPROMP system could potentially enable a more controlled film growth with high surface coverage and low roughness values. Furthermore, the addition of excess pyridine may prevent side reactions that lead to catalyst decomposition by reducing the instantaneous concentration and lifetime of the active species. The film thicknesses obtained after 25 h of CAPROMP using different concentrations of pyridine as an additive were determined by ellipsometry (Fig. 2a and Fig. S3 in ESI† for comparison by AFM scratch analysis). The results show an increase in thickness with pyridine concentration up to 100 mM (180 nm) and a significant decrease in thickness for high pyridine concentrations of 500 mM (80 nm) and 1000 mM (70 nm). Fig. 2b is a compilation of AFM images depicting the surface coverage of the films obtained with different pyridine concentrations after 25 h of CAPROMP. The films are relatively homogeneous for pyridine concentrations up to 100 mM, whereas at concentrations of 500 and 1000 mM, the surface coverage decreases significantly. This indicates that the reduction in catalyst activity not only restricts film growth to lower thicknesses, but also impairs homogeneous film formation. Therefore, by adding appropriate amounts of pyridine to the system, the transfer of catalyst in the assembly process is more efficient and catalyst lifetimes are prolonged, which is similar to that observed for solution polymerizations.32,34 However, at higher concentrations of pyridine, the equilibrium favors the rebinding of pyridine to the Ru carbene and the formation of the dormant species. Thus, the large reduction in catalyst activity inhibits film formation to a certain extent.
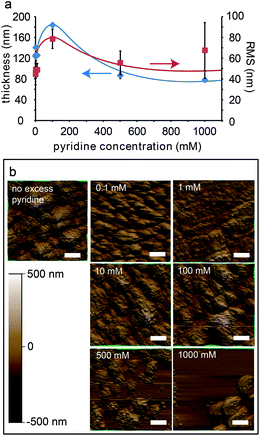 |
| Fig. 2 Effect of the addition of excess pyridine in CAPROMP using catalyst 3: (a) final film thickness (25 h), as determined by ellipsometry, and root mean square (RMS) surface roughness values determined by AFM versus pyridine concentration; and (b) 3D height-mode AFM images of P1 films obtained using various concentrations of pyridine (25 h). Scale bar = 1 μm. | |
Macrocross-linker parameters
Following on from the investigation into the effect of catalyst parameters, the influence of various macrocross-linker properties in the CAPROMP process was also studied, including macrocross-linker concentration, degree of pendent norbornene functionality and molecular weight.
Macrocross-linker concentration.
Fig. 3a and b illustrate the effect of different macrocross-linker concentrations (0.001, 0.01 and 1 mM) on the kinetics of film growth and surface topography, respectively. It was observed that the film formation rate and the subsequent final film thickness, as determined by ellipsometry, increased with higher macrocross-linker concentrations (Fig. 3a). The film thicknesses after 25 h of the CAP reaction were 120 nm (1 mM), 80 nm (0.01 mM) and 60 nm (0.001 mM). In addition, the surface coverage of the films was found to decrease with decreasing macrocross-linker concentration (Fig. 3b). The results are not surprising since higher macrocross-linker concentrations would also correlate to higher concentrations of polymerizable norbornene groups in the solution. Given that CAPROMP is a surface-confined polymerization process, increasing the monomer concentration would effectively increase the overall rate of polymerization (in this case, faster film growth). Based on these results, it can be concluded that a minimum macrocross-linker concentration of 1 mM is required to carry out an optimized CAPROMP reaction for the particular polymer and substrate employed in this study.
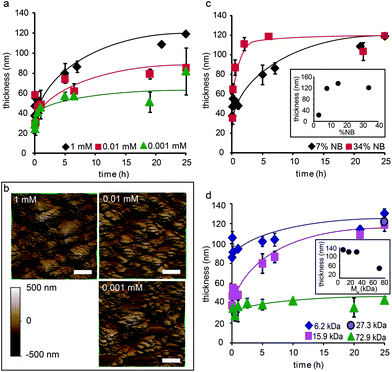 |
| Fig. 3 Effect of different macrocross-linker parameters on film properties: (a) the growth profile of CAP films versus time prepared from different concentrations of P1 using catalyst 3, as determined by ellipsometry; and (b) 3D height-mode AFM images of CAP films obtained from different concentrations of P1 using catalyst 3 after 25 h. (c) The growth profile of films versus time prepared using macrocross-linker P1 with various amounts of pendent norbornene groups, as measured by ellipsometry (inset depicts the final thickness of the films (at 25 h) prepared from macrocross-linkers consisting of different amounts of norbornenes). (d) Growth profiles of films versus time prepared using macrocross-linkers P1 with different molecular weights (inset depicts the final thickness (25 h) of the films prepared from macrocross-linkers with different molecular weights). Error bars: ±standard deviation (n = 3). Lines are to guide the eye. | |
Degree of pendent norbornene functionality.
For the CAP reaction to occur via ROMP, the number of pendent norbornene groups present on the macrocross-linker plays an important role, since polymerization of these groups allows one-step assembly and determines the degree of cross-linking of the resulting films. Therefore, the CAPROMP process was conducted using macrocross-linkers with various amounts of pendent norbornene groups (3, 7, 15 and 34 mol%). Analysis of the resulting films after 25 h by ellipsometry revealed that only relatively thick films (>100 nm) were obtained when the percentage of pendent norbornene groups in the polymer chain was ≥7 mol% (Fig. 3c, inset). For the macrocross-linker with 3 mol% pendent norbornene groups, only a thin film was obtained (ca. 30 nm), which may be related to the inefficient transfer of the active catalytic sites throughout the film and their ‘burial’ beneath polymer layers. For macrocross-linkers with >7 mol% norbornene moieties, the film growth was faster and more efficient. The rate of film growth with macrocross-linkers containing 34 mol% norbornene groups was observed to be significantly faster than that of films made with 7 mol% norbornene groups (Fig. 3c). This is attributed to the higher concentration and accessibility of the norbornene groups to react with the catalyst on the substrate and growing film. Therefore, macrocross-linkers approaching the surface have a higher probability of interacting with an active catalyst as a result of a higher concentration of norbornene groups and this in turn increases the rate of polymer deposition.
Molecular weight of macrocross-linker.
In the CAP approach, the molecular weight of the macrocross-linker is expected to play an important role, as it determines the rate of diffusion and hence the rate of reaction between the available norbornene groups and the active sites on the surface. In this study, several macrocross-linkers with different molecular weights were synthesized, while maintaining the same amount of pendent norbornene groups (7–9 mol%). Fig. 3d illustrates the evolution of film thickness as a function of time assembled using different molecular weights of macrocross-linker P1. A decrease in final film thickness was observed as the molecular weight of the macrocross-linker increased. This is expected since the pendent norbornene groups, which mediate the CAP reaction, are less accessible for large-sized polymers due to the random coil nature of the polymer. The accessibility of the pendent norbornenes is limited for the largest molecular weight macrocross-linker investigated (72.9 kDa), with a final film thickness of only 40 nm. Also, it is known that higher molecular weight polymers approach the surface at a slower rate.35,36 The diffusion rate of the polymers to the initiator-functionalized surface is more significant for the CAP films obtained at polymer molecular weights up to 27.3 kDa, where low molecular weight leads to faster kinetics with a similar final film thickness of 120 nm after 25 h (Fig. 3d). It should be noted that in this study, the different molecular weight macrocross-linkers have a nearly identical composition and the CAP process was conducted in dichloromethane, which is a good solvent for this type of polymer. Therefore, the intrinsic solubility and swelling characteristics, as well as solvation effects would be expected to be similar regardless of molecular weight. Hence, specifically for this system, the diffusion rate and norbornene accessibility are the two most important factors determining the film properties.
Conclusion
In this report, the influence of ROMP catalyst and macrocross-linker parameters on the properties of films fabricated by the CAPROMP approach was examined. Catalyst 3 was found to be the most efficient initiator for the CAPROMP system when using the poly(methyl methacrylate) macrocross-linker P1 in organic media. The addition of excess pyridine into the system up to a concentration of 100 mM was found to prolong the catalyst lifetime and consequently increase the film thickness and surface coverage. In addition, for the fabricated films to have high surface coverage, a minimum concentration of macrocross-linker P1 of 1 mM and 7 mol% pendent norbornene groups is required. Increasing the percentage of pendent norbornene groups resulted in an increase in the rate of film formation without increasing the final film thickness. Furthermore, increasing the molecular weight of the macrocross-linker decreased the rate of film formation and also the final film thickness. This study provides important insights into the parameters that influence the formation of films via the CAP approach and serves as a platform for the fabrication of the next generation of nanoscale films. In addition, the optimization of the CAPROMP approach will provide valuable insights into ways to tune other CAP systems, including those mediated via copper-mediated radical polymerization14 and photopolymerization.15 It is anticipated that optimization of the CAPROMP approach described here will provide access to tailored films for a diverse range of applications, including advanced multifunctional nanocoatings, biomaterials and separation technologies. Detailed examples of materials prepared by the CAP process for these applications will be reported in forthcoming publications.
Acknowledgements
The authors wish to acknowledge the Australian Research Council under the Federation Fellowship (FF0776078, F.C.), Future Fellowship (FT110100411, G.G.Q.) and Discovery Project (DP1094147, F.C., G.G.Q.) schemes for financial support of this work.
Notes and references
- S. F. M. Dongen, H. P. M. Hoog, C. J. H. Peters, M. Nallani, R. J. M. Nolte and J. C. M. Hest, Chem. Rev., 2009, 109, 6212 CrossRef.
- N. L. Rosi and C. A. Mirkin, Chem. Rev., 2005, 105, 1547 CrossRef CAS.
- R. Barbey, L. Lavanant, N. Paripovic, N. Schüwer, C. Sugnaux, S. Tugulu and H. A. Klok, Chem. Rev., 2009, 109, 5437 CrossRef CAS.
- J. Rühe and W. J. Knoll, J. Macromol. Sci., Part C, 2002, 42, 91 CrossRef.
- B. Zhao and W. J. Brittain, Prog. Polym. Sci., 2000, 25, 677 CrossRef CAS.
- G. Decher, Science, 1997, 277, 1232 CrossRef CAS.
- J. F. Quinn, A. P. R. Johnston, G. K. Such, A. N. Zelikin and F. Caruso, Chem. Soc. Rev., 2007, 36, 707 RSC.
- M. A. C. Stuart, W. T. S. Huck, J. Genzer, M. Müller, C. Ober, M. Stamm, G. B. Sukhorukov, I. Szleifer, V. V. Tsukruk and M. Urban, Nat. Mater., 2010, 9, 110 CrossRef.
- G. Liu, H. Zhang, X. Yang and Y. Wang, Polymer, 2007, 48, 5896 CrossRef CAS.
- G. Li, C. Lei, C. H. Wang, K. G. Neoh, E. T. Kang and X. Yang, Macromolecules, 2008, 41, 9487 CrossRef CAS.
- H. Lee, S. M. Dellatore, W. M. Miller and P. B. Messersmith, Science, 2007, 318, 426 CrossRef CAS.
- D. Mertz, P. Tan, Y. Wang, T. K. Goh, A. Blencowe and F. Caruso, Adv. Mater., 2011, 23, 5668 CrossRef CAS.
- T. K. Goh, S. N. Guntari, C. J. Ochs, A. Blencowe, D. Mertz, L. A. Connal, G. K. Such, G. G. Qiao and F. Caruso, Small, 2011, 7, 2863 CrossRef CAS.
- D. Mertz, C. J. Ochs, Z. Zhu, L. Lee, S. N. Guntari, G. K. Such, T. K. Goh, L. A. Connal, A. Blencowe, G. G. Qiao and F. Caruso, Chem. Commun., 2011, 47, 12601 RSC.
- E. H. H. Wong, S. N. Guntari, A. Blencowe, M. P. van Koeverden, F. Caruso and G. G. Qiao, ACS Macro Lett., 2012, 1, 1020 CrossRef CAS.
- M. S. Sanford, J. A. Love and R. H. Grubbs, Organometallics, 2001, 20, 5314 CrossRef CAS.
-
S. Michielsen, Polymer Handbook, 1999, 547 Search PubMed.
- P. D. Coffey, M. J. Swann, T. A. Waigh, F. Schedin and J. R. Lu, Opt. Express, 2009, 17, 10959 CrossRef CAS.
- C. W. Bielawski and R. H. Grubbs, Prog. Polym. Sci., 2007, 32, 1 CrossRef CAS.
- M. S. Sanford, J. A. Love and R. H. Grubbs, J. Am. Chem. Soc., 2001, 123, 6543 CrossRef CAS.
- C. W. Bielawski and R. H. Grubbs, Angew. Chem., Int. Ed., 2000, 39, 2903 CrossRef CAS.
- R. H. Grubbs, Tetrahedron, 2004, 60, 7117 CrossRef CAS.
- R. H. Grubbs, Angew. Chem., Int. Ed., 2006, 45, 3760 CrossRef CAS.
- J. A. Love, J. P. Morgan, T. M. Trnka and R. H. Grubbs, Angew. Chem., Int. Ed., 2002, 41, 4035 CrossRef CAS.
- S. B. Garber, J. S. Kingsbury, B. L. Gray and A. H. Hoveyda, J. Am. Chem. Soc., 2000, 122, 8168 CrossRef CAS.
- A. H. Hoveyda, D. G. Gillingham, J. J. V. Veldhuizen, O. Kataoka, S. B. Garber, J. S. Kingsbury and J. P. A. Harrity, Org. Biomol. Chem., 2004, 2, 8 CAS.
- J. S. Kingsbury, J. P. A. Harrity, P. J. Bonitatebus Jr and A. H. Hoveyda, J. Am. Chem. Soc., 1999, 121, 791 CrossRef CAS.
- A. Michrowska, R. Bujok, S. Harutyunyan, V. Sashuk, G. Dolgonos and K. Grela, J. Am. Chem. Soc., 2004, 126, 9318 CrossRef CAS.
- C. Larroche, J. P. Laval, A. Lattes and J. M. Basset, J. Org. Chem., 1984, 49, 1886 CrossRef CAS.
- J. D. Rule and J. S. Moore, Macromolecules, 2002, 35, 7878 CrossRef CAS.
- N. Seehof, S. Grutke and W. Risse, Macromolecules, 1993, 26, 695 CrossRef CAS.
- M. Ulman and R. H. Grubbs, J. Org. Chem., 1999, 64, 7202 CrossRef CAS.
- T. M. Trnka and R. H. Grubbs, Acc. Chem. Res., 2001, 34, 18 CrossRef CAS.
- C. W. Bielawski and R. H. Grubbs, Macromolecules, 2001, 34, 8838 CrossRef CAS.
- B. O'Shaughnessy and U. Sawhney, Macromolecules, 1996, 29, 7230 CrossRef CAS.
- H. Fredrickson, Phys. Rev. Lett., 1996, 76, 3440 CrossRef.
Footnote |
† Electronic supplementary information (ESI) available: Topography of CAPROMP films obtained using different ROMP catalysts and different catalyst concentrations, as imaged by AFM, and a comparison of CAPROMP film thickness by ellipsometry and AFM. See DOI: 10.1039/c2py20692g |
|
This journal is © The Royal Society of Chemistry 2013 |
Click here to see how this site uses Cookies. View our privacy policy here.