‘Clickable’ hydrogels for all: facile fabrication and functionalization†
Received
12th July 2013
, Accepted 30th July 2013
First published on 22nd August 2013
Abstract
In this study, we report a facile fabrication of poly(ethylene glycol) (PEG) based bulk and micro-patterned hydrogels that are amenable to functionalization with thiol-bearing molecules using the metal-free radical ‘thiol-ene’ click reaction. The hydrogels were synthesized using photopolymerization of commercially available monomers, namely allyl methacrylate and PEG-methacrylate in the presence of a PEG-dimethacrylate based crosslinker. Swelling behaviour of these hydrogels could be tailored by varying the amount of the hydrophilic monomer in the feed as well as varying the length of the PEG-chain in the methacrylate monomer. Importantly, the extent of functionalization of these hydrogels could be readily tuned by varying the amount of the reactive allyl methacrylate monomer. Analysis of nitrogen content in the hydrogels after conjugation of cysteamine demonstrated that the amount of cysteamine incorporation was in correlation with the amount of allyl groups in the hydrogels. Three-dimensional hydrogel patterns were fabricated using micromolding in capillaries. Tuneable conjugation of a thiol-containing dye molecule and a ligand-mediated immobilization of streptavidin onto these hydrogel patterns were realized. It was found that the swellability of the hydrogel patterns control the diffusion of streptavidin into the interior of the hydrogel matrix. These bio-inert hydrogels could be appended with peptides to promote cellular adhesion. Furthermore, it was demonstrated that the photochemical thiol-ene based method of conjugation enables localized attachment of thiol-containing molecules within these reactive hydrogels.
Introduction
The past decade has witnessed enormous advancement in the design and synthesis of novel functionalizable cross-linked hydrophilic polymeric materials, commonly referred to as hydrogels. The widespread interest in these materials stems from their prevalent applications in bio-medical and pharmaceutical sciences. In particular, some areas of their applications involve their use as a synthetic extracellular matrix,1,2 drug delivery systems,3 coating for implantable devices,4 biosensor platforms,5 separation systems6 and substrates for biomolecular immobilization.7 Oftentimes, the hydrogel matrix needs to be decorated with various small molecules or biomolecules to incorporate the functional attributes needed for the above mentioned applications.
Synthesis of hydrogels using photopolymerization has been a method of choice for several decades due to its operational simplicity and efficiency.8–10 Of course, the spatial and temporal control over polymerization, fast curing rates at ambient temperature, the requirement of a low solvent amount and minimal heat production are other desirable advantages of photopolymerization.11,12 It is important to note that for the widespread use of these materials by researchers who do not specialize in synthesis, the fabrication should be straightforward and pose minimal synthetic challenges. Utilization of readily available starting materials and synthesis of these hydrogels under non-stringent conditions without the use of any toxic metals and harsh reagents are highly desirable. Furthermore, hydrogels should be amenable to efficient post-polymerization modifications preferably using click chemistry.13–17
Post-modification of hydrogels under a mild and metal-catalyst free condition is highly desirable, especially for a functionalization involving sensitive biomolecules. The UV initiated radical thiol-ene click chemistry is generally a rapid reaction, free of side products, and does not require complex reagents or harsh reaction conditions. Due to these attributes, the thiol-ene reaction has been classified as a member of the click chemistry toolbox and has been used widely in polymer synthesis and modification.18–24 Additionally, the photochemically induced functionalization allows spatial control by selective UV irradiation.25 In light of these reasons, the utilization of thiol-ene reactions for both fabrication and functionalization of hydrogels is actively pursued.26–33 Recently, Anseth and co-workers reported synthesis of hydrogels using thiol-norbornene photopolymerization.31 They treated four-arm PEG bearing norbornene units at chain ends with dithiol-containing peptide linkers in the presence of a photoinitiator under UV-light.32 Additionally, these hydrogels were functionalized with thiol-containing peptides in a spatially controlled manner via a thiol-ene based photo-conjugation reaction.33 Synthesis of hydrogels with a PEG-based matrix has been actively pursued because of the inherent low affinity of PEG towards biomolecules and cells. Fabrication of PEG-based hydrogels containing reactive functional groups that enable efficient conjugation of desired ligands and biomolecules against a bio-inert background is desirable. To this end, recently Hawker and co-workers synthesized PEG-based hydrogels containing a microarray of reactive groups using the thiol-ene reaction.34 Various reactive groups such as azides, aldehydes and activated esters were embedded onto the surface of these hydrogels during the fabrication step to enable facile functionalization. As noted before, although much progress has been made in the design and fabrication of a variety of functionalizable hydrogels over the past few years; a lot remains to be done to improve the simplicity of fabrication and functionalization as well as their availability on a large scale, in a cost and effort efficient manner.
Patterned hydrogels have attracted considerable attention in recent years in micro array platforms for biomolecular immobilization, as well as substrates for cell growth. Recent reports demonstrate that the microstructure of the substrate also dictates cellular attachment, oriented immobilization and proliferation.35 Several studies demonstrate that micropatterned hydrogels can be obtained using a combination of soft lithography and photochemical techniques.36 Fabrication of reactive hydrogel micropatterns will provide an additional level of variability that can be tuned to modulate interaction of these substrates with the biological world.
In this work, a very simple and straightforward approach to fabricate thiol-reactive PEG-based bulk and micro-patterned hydrogels using photopolymerization is presented (Scheme 1). The allyl functional group is utilized as a reactive handle due to its facile conjugation to thiol-bearing molecules via photochemical thiol-ene reactions under mild and benign conditions. The amount of allyl group containing monomer in the feed helps tune the density of reactive groups within the hydrogel matrix, while the amount and nature of hydrophilic PEG-based monomer allow tuning of physical properties like swelling. Efficient post-polymerization functionalization of these hydrogels is carried out using the metal-free ‘thiol-ene’ click chemistry. The advantageous features of the photochemical based conjugation strategy to achieve spatially-addressable functionalization within the hydrogel matrix are also investigated.
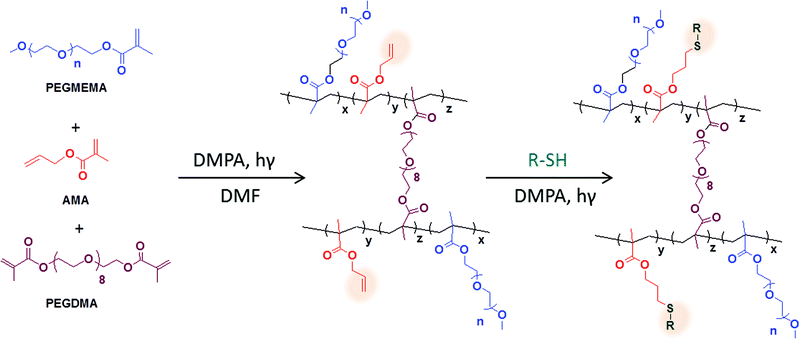 |
| Scheme 1 Schematic illustration of the synthesis and functionalization of thiol reactive hydrogels. | |
Experimental section
Materials
Allyl methacrylate (AMA) and poly(ethylene glycol) methyl ether methacrylate (PEGMEMA, Mn = 300 and Mn = 1100) and poly(ethylene glycol) dimethacrylate (PEGDMA, Mn = 550) were purchased from Sigma Aldrich and purified by passing through an activated aluminium oxide column prior to use. 2,2-Dimethoxy-2-phenylacetophenone (DMPA), 3-(trimethoxysilyl) propyl methacrylate (TMSMA), and cysteamine hydrochloride were purchased from Sigma Aldrich. N-Octadecyltrichlorosilane was obtained from ABCR GmbH & Co. KG. Nochromix was obtained from Godax Laboratories Inc. Biotinylated tri(ethylene glycol) undecane thiol (Biotin-SH) was purchased from Nanoscience Instruments (Phoenix, AZ). Fluorescein conjugated streptavidin (FITC-streptavidin) was obtained from Pierce. 4,4-Difluoro-1,3,5,7-tetramethyl-8-[(10-mercapto)]-4-bora-3a,4a-diaza-s-indacene (BODIPY-SH) was synthesized according to a literature procedure.37 Cyclic RGD thiol was synthesized according to the literature.38 Dimethylformamide was purchased from Lab-scan Analytical Sciences. Dichloromethane and methanol were purchased from Merck. Anhydrous toluene was obtained from a SciMatCo purification system. Column chromatography was performed using silica gel 60 (43–60 μm, Merck). Thin layer chromatography was performed using silica gel plates (Kieselgel 60 F254, 0.2 mm, Merck). The plates were viewed under a 254 nm UV lamp. All photopolymerization and thiol-ene reactions were performed at 365 nm using a handheld UV lamp (Blak-Ray UVP model B-100AP/R High Intensity UV lamp with a 100-watt spot bulb and 7° beam width). PDMS molds were fabricated using standard photolithographic techniques as described in the literature.39,40
Methods
Fabrication of bulk hydrogel. Representative procedure for the synthesis of hydrogel H1: A mixture of AMA (20 mg, 0.16 mmol), PEGMEMA (192 mg, 0.64 mmol), PEGDMA (11 mg, 0.02 mmol) and DMPA (5 mg, 0.02 mmol) in DMF (0.1 mL) in a small vial was sonicated for 30 seconds. The vial was placed under UV irradiation (365 nm) at a distance of 10 cm for 15 minutes. The hydrogel was washed several times with DMF followed by water to remove all unreacted reactants. The sample was frozen in water, and lyophilized. Other hydrogels were obtained similarly by varying the equivalents of the monomers AMA and PEGMEMA.
Swelling study of bulk hydrogels. A pre-weighed sample of lyophilized hydrogel was placed in a 25 mL beaker containing 10 mL of deionized water at room temperature. At regular intervals, the hydrogel sample was removed from the beaker and it was weighed after blotting on an absorbent tissue paper to eliminate excess adhered water. The water uptake was calculated using the following equation:
Wup = 100 × (Wwet − Wdry)/Wdry |
Wup refers to the water uptake, and Wwet and Wdry refer to the weight of wet and dry hydrogels respectively. Swelling experiments were repeated 3 times for a particular hydrogel.
Functionalization of bulk hydrogel. Cysteamine·HCl (8 mg), DMPA (4.5 mg) and MeOH were added to a vial and the mixture was sonicated for 30 seconds. Bulk hydrogel H1 (20 mg) was placed in the mixture and the vial was placed under UV irradiation (365 nm at a distance of 10 cm) for 30 minutes. The sample was washed several times with ethanol and water and then the bulk hydrogel was frozen in water and lyophilized. Other hydrogels were functionalized by varying the cysteamine HCl and DMPA amounts. Elemental analysis data were obtained using a Thermo Electron S.p.A Flash EA® 1112 elemental analyzer (CHNS separation column, PTFE: 2 m; 6 × 5 mm).
Modification of the glass surface for hydrogel adhesion. The glass surfaces were cleaned by sonicating in nochromix–sulphuric acid solution for 30 minutes. Thereafter, the glass surfaces were washed several times with deionized water, acetone and isopropyl alcohol and were dried in an oven. Thus cleaned glass surfaces were dipped in 1% solution of TMSMA in anhydrous toluene under a nitrogen atmosphere for 12 h. Glass surfaces were washed several times with toluene and then with methanol and they were dried under a gentle stream of nitrogen. Thus modified surfaces were stored under nitrogen.
Fabrication of patterned hydrogels. Polymer precursor solutions were prepared as described for the fabrication of bulk hydrogels. PDMS mold was carefully pressed onto a modified glass surface. A polymer precursor solution was dropped close to the open end of the PDMS mold. Immediately the channels were filled with a polymerization precursor solution by capillary action. Sample was placed under UV irradiation (365 nm) at the distance of 10 cm for 30 minutes. Irradiated samples were stored in a dark place for 12 h. Then the PDMS mold was gently peeled off the glass surface to reveal the hydrogel pattern. The patterned surface was washed with copious amounts of DMF or CH2Cl2 to remove unreacted monomers.
Functionalization of patterned hydrogel. BODIPY-SH, DMPA and THF were added to a vial, and the mixture was sonicated for 30 seconds. The mixture was dropped onto a hydrogel pattern and the sample was placed under UV irradiation (365 nm) at the distance of 10 cm for 5 minutes. After the reaction, the sample was washed several times with THF to remove unreacted reagents.
Fluorescence microscopy. Attachment of fluorescent dye molecules (BODIPY-SH) and fluorescently labelled enzyme (streptavidin) was determined using an LD-A-Plan 10×/0.30 objective in a Zeiss Axio Observer inverted microscope (Zeiss Fluorescence Microscopy, Carl Zeiss Canada Ltd, Canada). A Zeiss Filter set 38 (Excitation BP 470/40, Emission BP 525/50) was used for imaging the functionalized hydrogel surface. For visualizing cells on the surface, a Zeiss Filter set 49 (Excitation G365, Emission BP 445/50) was used for imaging DAPI stained nuclei. Images were processed using Zeiss AxioVision software. Fluorescent and phase contrast images were merged to visualize the cell nuclei and cellular morphology, respectively. Images were processed using Zeiss AxioVision software.
Biofunctionalization of patterned hydrogel. A solution of Biotin-SH (10 mg in 385 μL MeOH) and DMPA (1.170 mg in 50 μL MeOH) was mixed and dropped onto a dried hydrogel pattern. The sample was placed under UV irradiation (365 nm) at the distance of 10 cm for 30 minutes. After the reaction, the sample was washed with MeOH and dried. A solution of FITC-streptavidin (20 μL, 0.1 mg mL−1 in H2O) was dropped on the biotinylated hydrogel sample. The pattern was placed overnight in a dark place, and then the hydrogel sample was gently rinsed in copious amounts of water.
Fabrication of the hydrophobic glass surface. Glass surfaces were cleaned by sonicating in nochromix–sulphuric acid solution for 30 minutes and then washed several times with deionized water, acetone and isopropyl alcohol and then dried in oven. Cleaned glass surfaces were dipped in 1% solution of n-octadecyltrichlorosilane in anhydrous toluene overnight. Surfaces were washed several times with toluene and then with methanol and they were dried under a nitrogen stream.
Fabrication of hydrogel layers on the glass surface. A polymer precursor solution (20% AMA with PEGMEMA Mn = 300) was prepared as described in the fabrication of bulk hydrogels. A polymer precursor solution was spin-coated on a methacrylate modified glass surface in two steps: first at 500 rpm for 4 seconds, followed by a second step at 800 rpm for 30 seconds. After spin-coating a hydrophobic glass surface was placed on a thin layer of hydrogel and the sample was placed under UV irradiation (365 nm at the distance of 10 cm) for 30 minutes. The hydrophobic glass was gently peeled off after a few hours and the sample was washed several times with CH2Cl2.
Photo-patterned functionalization of hydrogels. Hydrogels on glass slides were functionalized via a thiol-ene reaction using a thiol-containing dye, namely BODIPY-SH. A mixture of BODIPY-SH and DMPA in THF was dropped on the hydrogel film and a TEM grid was firmly placed onto the hydrogel surface. The sample was placed under UV irradiation (365 nm at the distance of 10 cm) for 5 minutes. After the reaction the TEM grid was gently peeled off and the sample was washed several times with THF to extract any unreacted dye.
Cyclic RGD-SH immobilization on hydrogel layers on a glass surface. 10 μL RGD-SH (0.5 mg mL−1) and 10 μL of DMPA solution (0.0885 mg mL−1) in DMF were mixed and spread over a 2 cm2 hydrogel layer on a glass slide. Sample was placed under UV irradiation (365 nm at the distance of 5 cm) for 10 minutes. The hydrogel sample was washed with DMF three times and sonicated in DMF for 10 minutes to remove any unbound peptide. Then hydrogels were washed with MeOH and water and swollen in water overnight. Control samples were incubated with peptide in the absence of UV irradiation and a similar washing procedure was utilized.
Cell culture on hydrogels. Mouse fibroblast L929 cells were routinely maintained in DMEM high glucose medium supplemented with 10% FBS, 1% nonessential amino acids, 4 mM L-glutamine, penicillin (100 U mL−1) and streptomycin (100 μg mL−1), in a humidified chamber under 5% CO2 at 37 °C. Cells were harvested with 0.05% Trypsin–EDTA treatment and counted via a Cedex Cell Counter (Roche Applied Science) using a trypan blue dye exclusion test. 2 × 105 mL−1 cells were resuspended in DMEM medium containing 1% FBS and seeded on previously sterilized hydrogel surfaces in 6-well plates. Following incubation for 4 hours, cell culture media were removed; cells were washed 3 times with PBS and fixed using 4% formaldehyde for 15 minutes. Cell nuclei were stained with 4′,6-diamidino-2-phenylindole (DAPI) (1 μg mL−1 in MilliQ water) for 10 minutes at room temperature.
Results and discussion
Bulk hydrogels
A library of bulk hydrogels (H1–H6) was synthesised by either varying the ratio of reactive monomer AMA to the hydrophilic monomer PEGMEMA or varying the chain length of the PEGMEMA monomer. Hydrogels were prepared using a simple protocol involving mixing of the monomers and free radical photopolymerization under UV irradiation (365 nm), in the presence of DMPA as a photo-initiator and poly(ethylene glycol) dimetharcylate as a crosslinker. The hydrogel compositions and conversions of gelation are tabulated in Table 1. The hydrogels obtained by photopolymerization were transparent and soft. All hydrogels exhibited a good swelling capacity due to the presence of hydrophilic monomer. For a similar monomer composition, hydrogels fabricated with less hydrophilic PEG-containing monomer (H1–H3) exhibited a lower extent of swelling than hydrogels (H4–H6) containing the more hydrophilic PEG-based monomer (Fig. 1). Furthermore, both sets of hydrogels show a decrement of water uptake capability with increasing amount of hydrophobic monomer. Microstructures of these bulk hydrogels were investigated by scanning electron microscopy (SEM) (Fig. 2). The hydrogels did not exhibit a sponge-like porous morphology but had a rather rubber like appearance.
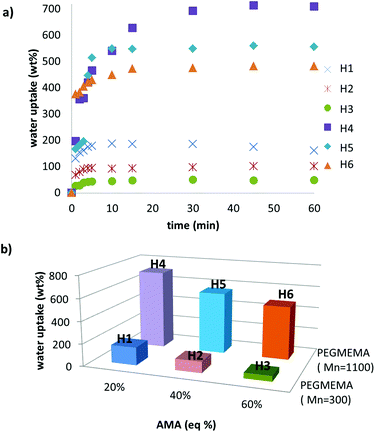 |
| Fig. 1 (a) Water uptake plots and (b) comparison of water uptake capabilities with the variation of monomer ratio and chain length of PEG. | |
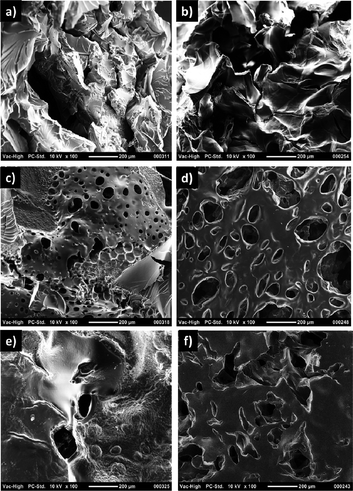 |
| Fig. 2 Representative SEM images of hydrogels (a) H1, (b) H3, (c) H2, (d) H4, (e) H5 and (f) H6. | |
Table 1 Synthesis of bulk-hydrogels using photopolymerization
Samplea |
AMA : PEGMEMAb |
PEGMEMAc (Mn) |
Gel contentd (%) |
Bulk hydrogels. Mole ratio of monomers. Molecular weight of PEG monomer. Calculated as (dry hydrogel weight/total weight of monomer and crosslinker) × 100. |
H1 |
20 : 80 |
300 |
82 |
H2 |
40 : 60 |
300 |
87 |
H3 |
60 : 40 |
300 |
85 |
H4 |
20 : 80 |
1100 |
49 |
H5 |
40 : 60 |
1100 |
68 |
H6 |
60 : 40 |
1100 |
72 |
The bulk hydrogels were analyzed by infrared (IR) spectroscopy to characterize the increasing amount of allyl groups in the hydrogels. As expected, the IR spectra of the hydrogels exhibit an increase in the intensity of the double bond stretching peak at 1638 cm−1 due to the increment of allyl group with an increase in the amount of AMA monomer (see ESI†). It must be noted that consumption of some of the allyl groups during the radical polymerization cannot be completely ruled out. Additionally, ‘thiol-ene’ functionalization of the bulk gels (H1–H3) with nitrogen containing thiol-based ligand, namely cysteamine, was carried out. Hydrogels were conjugated with cysteamine in the presence of DMPA under UV irradiation. Elemental analysis of thus functionalized hydrogels showed a proportional increment of nitrogen content with the increase of allyl groups in the hydrogels (Fig. 3).
Patterned hydrogels
Three-dimensional micropatterns of hydrogels were fabricated using the micro-molding in capillaries (MIMIC) technique.38 The technique involves spontaneous filling of the channels of a PDMS mold by a polymeric precursor solution, followed by photopolymerization under UV illumination to produce micro-patterned hydrogels (Fig. 4a).
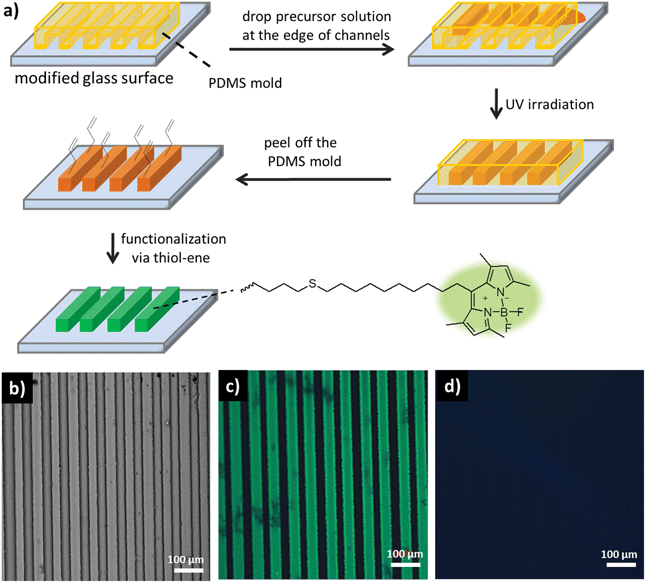 |
| Fig. 4 (a) Hydrogel micropattern fabrication via MIMIC and functionalization with BODIPYSH under UV irradiation. (b) Optical microscope image of patterned hydrogel H1. Fluorescence microscope images illustrating (c) BODIPYSH attachment to hydrogel H1 under UV irradiation and (d) no BODIPYSH attachment to hydrogel H1 in the absence of UV irradiation. | |
Employing the MIMIC technique to photopolymerize hydrogel precursors containing the AMA and PEGMEMA monomers, well-defined micropatterns were readily obtained as evident from the optical micrograph (Fig. 4b). These micropatterns could be easily functionalized via thiol-ene reaction of a thiol-containing ligand with the allyl functional groups in the gels. A thiol-containing fluorescent dye, namely BODIPY-SH, was chosen for attachment (Fig. 4). The hydrogel patterns containing the dye in the presence of DMPA were irradiated with UV light. Fluorescence microscopy analysis of thus functionalized patterns revealed successful attachment of the dye (Fig. 4c). A control experiment carried out under similar conditions but devoid of any UV exposure revealed negligible functionalization (Fig. 4d).
These hydrogels present the ability to tailor the amount of immobilization of desired biomolecules on these polymeric platforms. Higher extent of functionalization can be expected for hydrogels containing more allyl moieties. As noted before, the amount of reactive allyl functional groups within the hydrogels can be easily tailored by adjusting the stoichiometry of the monomers in the feed. Evaluation of the efficiency of ligand-mediated bioimmobilization on these hydrogel patterns in a controlled manner was undertaken. Hydrogels with varying amounts of allyl group (H1–H3) were functionalized with a biotin-based thiol-containing ligand (Biotin-SH) using the thiol-ene conjugation. Biotin is a ligand that is known to have a very high affinity for the protein streptavidin.41 Biotinylated hydrogels were exposed to a FITC-streptavidin containing solution. As expected, hydrogels with higher content of functionalization handles, i.e. the allyl groups, contained higher amounts of streptavidin (Fig. 5). Interestingly, analysis of a sample cross-section of hydrogel H3 using confocal fluorescence microscopy revealed that far more functionalization takes place on the surface of these gels than their interiors. This happens due to the lower swellability of the gels prepared with shorter PEG-chain bearing monomer, resulting in slow diffusion of the large protein molecules. Indeed, the hydrogel patterns (H6) with higher swelling, fabricated using more hydrophilic monomer with longer PEG chains, enables homogeneous protein immobilization, as revealed by the uniform fluorescence of the entire cross-section (Fig. 6).
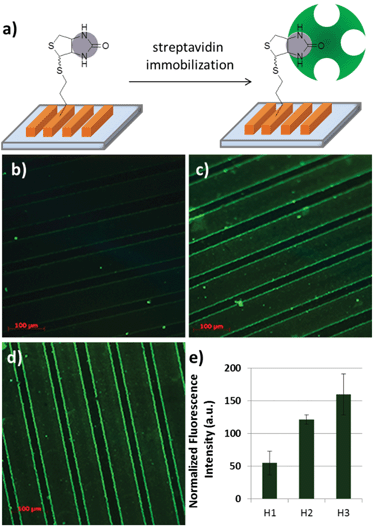 |
| Fig. 5 (a) FITC labelled streptavidin immobilization on biotinylated hydrogels. Fluorescence microscope images of (b) H1, (c) H2 and (d) H3 patterns and (e) relative fluorescence intensities. | |
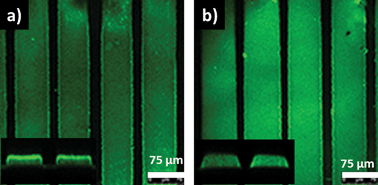 |
| Fig. 6 Confocal fluorescence microscope images of streptavidin immobilized photo-patterned hydrogels (a) H3 and (b) H6. Fluorescence along the cross-section as shown in the insets. | |
Adhesion of particular cell types onto hydrogel scaffolds can be increased by their modification with peptide fragments that have an affinity for the receptors on cell surfaces. To demonstrate the minimal non-specific cellular attachment and efficient specific attachment, the allyl-functionalized hydrogels were covalently conjugated with cyclic peptides containing the RGD-sequence, a peptide motif known to bind to the αvβ3 integrin receptors found on certain cell surfaces. A thiol containing cRGDfC was conjugated to the allyl groups using the thiol-ene chemistry. Fibroblasts, a cell type known to have affinity for this particular peptide sequence, were seeded onto unmodified and modified surfaces. While there was minimal attachment of cells on hydrogels devoid of any peptides, cells attached onto the RGD-containing hydrogels (Fig. 7). This demonstrates that these anti-biofouling surfaces can be easily modified with ligands to promote the binding of cells of interest.
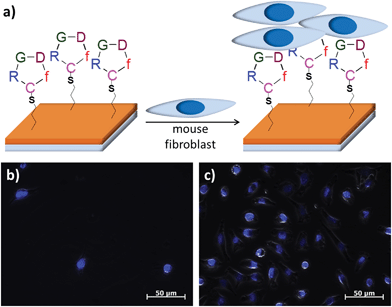 |
| Fig. 7 (a) Schematic of mouse fibroblast immobilization on RGD functionalized hydrogels. Mouse fibroblast L929 cells on (b) non-RDG and (c) RGD-bound hydrogels. | |
An additional advantage of photochemically induced conjugation reactions is that they enable attachment of desired molecules in a spatially controlled manner by using selective illumination through a photomask. To demonstrate this, a layer of hydrogel was swollen in a solution of BODIPY-SH and the photoinitiator DMPA, and then illuminated through a TEM grid to allow thiol-ene reactions only in the exposed areas (Fig. 8a). The sample was washed with copious amounts of a solvent to remove any unbound dye. Analysis of thus modified hydrogels using confocal fluorescence microscopy revealed that the dye attachment had occurred only in the UV-exposed areas through the entire depth of the hydrogel matrix (Fig. 8b and 8c).
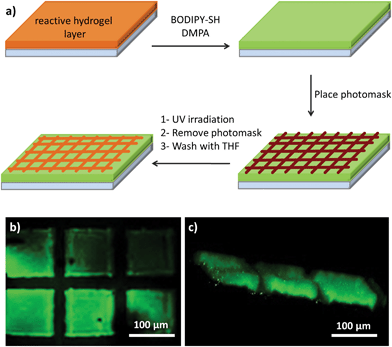 |
| Fig. 8 Confocal fluorescence microscope images of FITC-streptavidin immobilized (a) H3 and (b) H6 patterns and (c) their cross-sections as insets. | |
Conclusions
Bulk and patterned hydrogels containing varying amounts of the allyl-functional group were prepared under UV irradiation in the presence of a photo-initiator (DMPA) and a crosslinker (PEGDMA). Water uptake capacity could be tuned by adjusting the amount and chain length of the PEG-based comonomer. Patterned hydrogels were fabricated by using the MIMIC technique. These hydrogels were functionalized via the thiol-ene ‘click’ chemistry using thiol containing molecules. The degree of functionalization of these hydrogels can be tailored by varying the amount of reactive allyl groups within the hydrogels. The effect of the swellability of the hydrogels on the functionalization within the hydrogel matrix was investigated. Confocal microscopy images of the cross-section of hydrogel patterns revealed that a homogeneous functionalization is obtained for the more swellable hydrogels. It was demonstrated that these hydrogels have minimal non-specific cell adhesion, but can be modified easily with thiol containing peptide ligands to promote cellular adhesion. Positionally controlled conjugation of thiol-containing molecules onto these hydrogel platforms was demonstrated by conducting the thiol-ene reaction using a photo-mask. It is envisaged that the simplicity in fabrication as well as the facile and efficient functionalization of these hydrogels will promote their usage in biomolecular immobilization.
Acknowledgements
Authors thank Prof. Stefan Fuss for assistance with the confocal microscope and Prof. Senol Mutlu for assistance with plasma cleaner and stamp preparation. The cell line used in this study was kindly provided by Dr Nesrin Ozoren from Bogazici University, Molecular Biology and Genetics Department. R. S. thanks the Turkish Academy of Sciences for financial assistance through a TUBA-GEBIP fellowship. R. S. thanks the state planning commission of Turkey for financial assistance under DPT grant no. 09K120520. T. N. G. thanks the Bogazici University Foundation (BUVAK) for a fellowship.
Notes and references
- M. W. Tibbitt and K. S. Anseth, Biotechnol. Bioeng., 2009, 103, 655 CrossRef CAS PubMed.
- J. L. Drury and D. J. Mooney, Biomaterials, 2003, 24, 4337 CrossRef CAS.
- N. A. Peppas, K. B. Keys, M. Torres-Lugo and A. M. Lowman, J. Controlled Release, 1999, 62, 81 CrossRef CAS.
- S. Rahimi, E. H. Sarraf, G. K. Wong and K. Takahata, Biomed Microdevices, 2011, 13, 267 CrossRef CAS PubMed.
- G. Justin, S. Finley, A. R. Abdur Rahman and A. Guiseppi-Elie, Biomed Microdevices, 2009, 11, 103 CrossRef CAS PubMed.
- Y. J. Choi, T. Yamaguchi and S. Nakao, Ind. Eng. Chem. Res., 2000, 39, 2491 CrossRef CAS.
- Y. Luo and M. S. Shoichet, Biomacromolecules, 2004, 5, 2315 CrossRef CAS PubMed.
- K. S. Anseth and J. A. Burdick, MRS Bull., 2002, 27, 130 CrossRef CAS.
- D. M. Marsden, R. L. Nicholson, M. Ladlow and D. R. Spring, Chem. Commun., 2009, 7107–7109 RSC.
- G. Yilmaz, M. U. Kahveci and Y. Yagci, Macromol. Rapid Commun., 2011, 32, 1906 CrossRef CAS PubMed.
- Y. Yagci, S. Jockusch and N. J. Turro, Macromolecules, 2010, 43, 6245 CrossRef CAS.
- K. T. Nguyen and J. L. West, Biomaterials, 2002, 23, 4307 CrossRef CAS.
- K. Kempe, A. Krieg, C. R. Becer and U. S. Schubert, Chem. Soc. Rev., 2012, 41, 176 RSC.
- W. Van Camp, T. Dispinar, B. Dervaux, F. E. Du Prez, J. C. Martins and B. Fritzinger, Macromol. Rapid Commun., 2009, 30, 1328 CrossRef CAS PubMed.
- H. Altin, I. Kosif and R. Sanyal, Macromolecules, 2010, 43, 3801 CrossRef CAS.
- C. R. Becer, R. Hoogenboom and U. S. Schubert, Angew. Chem., Int. Ed., 2009, 48, 4900 CrossRef CAS PubMed.
- I. Kosif, E. J. Park, R. Sanyal and A. Sanyal, Macromolecules, 2010, 43, 4140 CrossRef CAS.
- A. B. Lowe, Polym. Chem., 2010, 1, 17 RSC.
- H. Durmaz, M. Butun, G. Hizal and U. Tunca, J. Polym. Sci., Part A: Polym. Chem., 2012, 50, 3116 CrossRef CAS.
- I. I. Yilmaz, M. Arslan and A. Sanyal, Macromol. Rapid Commun., 2012, 33, 856 CrossRef CAS PubMed.
- C. E. Hoyle and C. N. Bowman, Angew. Chem., Int. Ed., 2010, 49, 1540 CrossRef CAS PubMed.
- M. J. Kade, D. J. Burke and C. J. Hawker, J. Polym. Sci., Part A: Polym. Chem., 2010, 48, 743 CrossRef CAS.
- A. Pacini, M. Caricato, S. Ferrari, D. Capsoni, A. Martínez de Ilarduya, S. Munoz-Guerra and D. Pasini, J. Polym. Sci., Part A: Polym. Chem., 2012, 50, 4790 CrossRef CAS.
- K. Kempe, T. Neuwirth, J. Czaplewska, M. Gottschaldt, R. Hoogenboom and U. S. Schubert, Polym. Chem., 2011, 2, 1737 RSC.
- V. S. Khire, A. W. Harant, A. W. Watkins, K. S. Anseth and C. N. Bowman, Macromolecules, 2006, 39, 5081 CrossRef CAS.
- V. X. Truong, I. A. Barker, M. Tan, L. Mespouille, P. Dubois and A. P. Dove, J. Mater.
Chem. B, 2013, 1, 221 RSC.
- S. Yigit, R. Sanyal and A. Sanyal, Chem.–Asian J., 2011, 6, 2648 CrossRef CAS PubMed.
- T. R. Dargaville, R. Forster, B. L. Farrugia, K. Kempe, L. Voorhaar, U. S. Schubert and R. Hoogenboom, Macromol. Rapid Commun., 2012, 33, 1695 CrossRef CAS PubMed.
- T. Yang, H. Long, M. Malkoch, E. K. Gamstedt, L. Berglund and A. Hult, J. Polym. Sci., Part A: Polym. Chem., 2011, 49, 4044 CrossRef CAS.
- A. A. Aimetti, A. J. Machen and K. S. Anseth, Biomaterials, 2009, 30, 6048 CrossRef CAS PubMed.
- B. D. Polizzotti, B. D. Fairbanks and K. S. Anseth, Biomacromolecules, 2008, 9, 1084 CrossRef CAS PubMed.
- J. D. McCall and K. S. Anseth, Biomacromolecules, 2012, 13, 2410 CrossRef CAS PubMed.
- B. D. Fairbanks, M. P. Schwartz, A. E. Halevi, C. R. Nuttelman, C. N. Bowman and K. S. Anseth, Adv. Mater., 2009, 21, 5005 CrossRef CAS.
- N. Gupta, B. F. Lin, L. M. Campos, M. D. Dimitriou, S. T. Hikita, N. D. Treat, M. V. Tirrell, D. O. Clegg, E. J. Kramer and C. J. Hawker, Nat. Chem., 2010, 2, 138 CrossRef CAS PubMed.
- C. Subramani, N. Cengiz, K. Saha, T. N. Gevrek, X. Yu, Y. Jeong, A. Bajaj, A. Sanyal and V. M. Rotello, Adv. Mater., 2011, 23, 3165 CrossRef CAS PubMed.
- D. B. Weibel, W. R. DiLuzio and G. M. Whitesides, Nat. Rev. Microbiol., 2007, 5, 209 CrossRef CAS PubMed.
- J. L. Shepherd, A. Kell, E. Chung, C. W. Sinclar, M. S. Workentin and D. Bizzotto, J. Am. Chem. Soc., 2004, 126, 8329 CrossRef CAS PubMed.
- C. F. McCusker, P. J. Kocienski, F. T. Boyle and A. G. Schatzlein, Bioorg. Med. Chem. Lett., 2002, 12, 547 CrossRef CAS.
- T. N. Gevrek, R. N. Ozdeslik, G. S. Sahin, G. Yesilbag, S. Mutlu and A. Sanyal, Macromol. Chem. Phys., 2012, 213, 166 CrossRef CAS.
- E. Kim, Y. Xia and G. M. Whitesides, J. Am. Chem. Soc., 1996, 118, 5722 CrossRef CAS.
- J. Moss and M. D. Lane, Adv. Enzymol. Relat. Areas Mol. Biol., 1971, 35, 321 CAS.
|
This journal is © The Royal Society of Chemistry 2014 |
Click here to see how this site uses Cookies. View our privacy policy here.