DOI:
10.1039/C4BM00200H
(Review Article)
Biomater. Sci., 2014,
2, 1562-1573
The instructive role of the vasculature in stem cell niches
Received
3rd June 2014
, Accepted 29th July 2014
First published on 6th August 2014
Abstract
An important hallmark of many adult stem cell niches is their proximity to the vasculature in vivo, a feature common to neural stem cells (NSCs), mesenchymal stem cells (MSCs) from bone marrow, adipose, and other tissues, hematopoietic stem cells (HSCs), and many tumor stem cells. This review summarizes key studies supporting the vasculature's instructive role in adult stem cell niches, and the putative underlying molecular mechanisms by which blood vessels in these niches exert control over progenitor cell fates. The importance of the perivascular niche for pathology, notably tumor metastasis and dormancy, is also highlighted. Finally, the implications of the perivascular regulation of stem and progenitor cells on biomaterial design and the impact on future research directions are discussed.
 Andrew J. Putnam | Andrew Putnam is an associate professor in the Department of Biomedical Engineering and Director of the Cellular Biotechnology Training Program at the University of Michigan. The extracellular matrix is the central focus of his laboratory, with an emphasis on cell/ECM-based strategies to direct vascularization. Dr Putnam's research has been funded by the National Institutes of Health, National Science Foundation, California Institute for Regenerative Medicine, American Heart Association, and other foundations. He is a recipient of the NSF CAREER Award (2007) and a New Faculty Award from CIRM (2008), and plays a very active role in the educational mission of his department. |
Introduction
Cell-based regenerative medicine approaches offer the potential to transform current clinical treatments for many diseases, disabilities, and traumatic injuries. However, successful implementation of such approaches hinges on the ability to predictably and reliably control cell fate decisions. In the body, such decisions are governed not only via genetic and epigenetic means (i.e., cell intrinsic), but also by many different microenvironmental features (i.e., cell extrinsic), including soluble and insoluble morphogens, cues from other cells, and the extracellular matrix (ECM). Collectively, these factors constitute the stem cell niche, which helps maintain stem cell quiescence by regulating self-renewal and promotes their ability to support tissue homeostasis by controlling differentiation.
The concept of the stem cell niche was first proposed by Schofield with respect to HSCs, and refers to a specialized microenvironment that includes supporting cells along with their secreted trophic factors that influence stem cell phenotype.1 The numerous aforementioned instructive cues that comprise post-natal adult stem cell niches coordinately regulate cell fate decisions with precise spatiotemporal control.2,3 Furthermore, there is evidence that the intrinsic mechanical properties of the ECM influence cell fate decisions in MSCs,4 NSCs,5 and skeletal muscle stem cells.6 However, the complexity and integration of these various elements remains poorly understood. Artificial stem cell niches may augment efforts to identify the specific cues that define stem cell niches, and thereby pave the way for the successful use of stem cells in regenerative medicine.7–9 While complete recapitulation of stem cell microenvironments ex vivo has yet to be achieved, an improved understanding of in vivo niches will greatly facilitate this goal.
The development of artificial stem cell niches has accelerated in the bioengineering community in recent years.10–12 The most common current paradigm involves the use of information-rich, “instructive” biomaterials, which could conceivably direct cell fate in vitro and provide a template for tissue formation in vivo.11 These materials are often designed with tunable mechanical, chemical, cell-adhesive, and/or geometric or topographic properties to achieve maximal control of cell fate. Various permutations of this approach have been highlighted in several other reviews,2,11,13 and there is wide consensus that these approaches will continue to be important avenues of investigation.
Several recent studies suggest that another feature common to many adult stem niches is critically important in the regulation of cell fate: proximity to the vasculature.14 This anatomic location, the so-called perivascular niche, has been suggested as the in vivo location of adult NSCs,15–17 MSCs from bone marrow and multiple other adult tissues,18 and HSCs.19 Interactions between stem cells and endothelial cells (ECs) in the niche are reciprocal and dynamic, and current knowledge of the molecular basis of these interactions is in its infancy. A more complete understanding of these interactions and the nature of their regulation offer exciting potential to incorporate signals found in the vascular niche into synthetic microenvironments. However, even as more molecular players are identified, it is conceivable that combinations of peptide- or protein-functionalized biomaterials, soluble morphogens (or gradients thereof), or physical cues may not fully reproduce the perivascular interface between adult stem cells and the vasculature. Instead, the anatomic structure of an intact blood vessel may be an essential and instructive part of the niche.
In this review, I will summarize key papers in the literature that have identified the perivascular space as an important niche component of NSCs, MSCs, and HSCs, highlighting the status of these populations for clinical use. Recent evidence that dormant tumor cells with stem cell-like properties reside in instructive perivascular niches will also be described, followed by a section highlighting some of the known molecular regulators within the perivascular niche. I will also discuss strategies to recreate the perivascular niche in vitro and in vivo, and the potential to use biomaterials to mimic key aspects of the niche both for fundamental discovery and therapeutics. Finally, the review will close with a brief summary of the implications of perivascular niches and opportunities for future directions.
The perivascular niche of neural stem cells
As their name implies, NSCs are a population of progenitor cells present in the central nervous system that give rise to neurons, astrocytes, and oligodendrocytes.20,21 The discovery of NSCs is typically attributed to a 1989 paper by Temple, who isolated dividing cells from embryonic day 13.5–14.5 rat forebrains and demonstrated that these cells can differentiate into other clonal types.22 Over the past 20 years, critical studies have demonstrated that NSCs generate neurons throughout life (at least in mice) in the forebrain subventricular zone (SVZ) and the hippocampal dentate gyrus, giving rise to subpopulations of transit-amplifying cells and neuroblasts.21,23 These cells are of high interest to both the neurobiology and regenerative medicine communities: to the former because of the fundamental organogenesis questions associated with neurodevelopmental and neurodegenerative diseases, and to the latter because of the potential to use these cells to cure CNS disorders like amyotrophic lateral sclerosis (ALS), Alzheimer's disease, and Parkinson's disease. NSCs are the focus of several ongoing human clinical trials, the first of which is for patients with ALS (see ClinicalTrials.gov). Phase 1 of this trial began in 2011, and the company (Neuralstem, Inc.) behind the new therapy recently began recruiting patients for Phase 2; a total of 29 patients have been treated in the trial so far. Neuralstem recently launched a new trial for the use of NSCs to treat patients with spinal cord injuries as well. StemCells, Inc., another company focused on NSC-based therapies, has injected their cells in human patients in both Europe and Canada, with the goal of remyelinating axons damaged by injury or disease (i.e., functioning as oligodendrocytes). Another ongoing clinical trial is focused on the use of NSCs in the treatment of patients who have suffered ischemic strokes. Fetal tissue is the source of the human NSCs for these clinical trials.
The location of neurogenesis in the adult mammalian hippocampus was first identified as an anatomically contiguous co-association of newly generated neurons with ECs.24 Studies in other model systems demonstrated that angiogenesis and neurogenesis are spatially and temporally linked.25 Subsequent efforts used an in vitro co-culture system to examine soluble factor cross-talk between ECs and NSCs, and demonstrated that EC-derived cues are able to stimulate NSC self-renewal and enhance their abilities to differentiate into neurons.15 However, perhaps the most compelling evidence that NSCs occupy a perivascular niche in vivo appeared in two papers published in 2008,16,17 both of which performed careful and comprehensive histological analyses of whole mount and sectioned mouse forebrains. Tavazoie et al. demonstrated that the NSCs and their closely related progeny are tightly apposed to the blood vessels in the SVZ, with the transit-amplifying progeny (the so-called C cells) often contacting the vasculature directly at sites where the blood-brain barrier is incomplete (i.e., in regions with sparse astrocyte and pericyte coverage).17 Shen et al. similarly showed that NSCs and their progeny in the SVZ of mouse forebrains are closely associated with an extensive vascular plexus, and further revealed that a specific integrin–laminin interaction (discussed further below) is essential for the localization of the progenitor cells with the vasculature.16 Collectively, these studies have led to a fairly detailed conceptual view of the perivascular niche for NSCs (Fig. 1).
 |
| Fig. 1 Perivascular niche of neural stem cells. This cartoon illustrates the architecture of the perivascular niche of NSCs in the adult mammalian subventricular zone. It shows the relationships between the NSCs (the so called “B” cells) and their progeny, the transit-amplifying “C” cells and neuroblastic “A” cells. The schematic demonstrates the proximity of the NSC population to the vasculature, and also highlights several candidate soluble and insoluble interactions. Reprinted by permission from Macmillan Publishers Ltd: Nature Neuroscience,21 copyright 2011. | |
Recent studies have revealed that the perivascular niche of NSCs, and the identity of NSCs themselves, is more complex. In particular, Codega et al. identified two distinct populations of NSCs within the SVZ, one that is quiescent and one that is activated.26 The quiescent NSCs were determined to be GFAP+, CD133+, and Nestin-, whereas the activated NSCs upregulate Nestin and the epidermal growth factor receptor (EGFR). Further screening of the transcriptomes of these two populations of NSCs showed that sphingosine-1-phosphate (S1P) and prostaglandin D2 (PGD2) could inhibit the activation of the quiescent NSCs. Both of these molecules are found in cerebrospinal fluid, but may also be circulating within the vasculature. Thus, whether the vasculature instructively contributes to the quiescence of NSCs remains to be seen.
The perivascular niche of mesenchymal stem cells
Mesenchymal stem cells (MSCs) are a population of adult tissue-derived adherent cells that were first discovered in the bone marrow of the iliac crest by Friedenstein et al.27,28 These cells were initially identified based on their ability to form clonal adherent colonies of fibroblastic cells (“colony forming unit-fibroblast”), and later shown to possess the capacity to differentiate into bone, cartilage, and fat.29 This latter capability is why they were initially dubbed “stem” cells.30 According to the Mesenchymal and Tissue Stem Cell Committee of the International Society for Cellular Therapy (ISCT), the criteria which define MSCs include: plastic adherence following plating in 2D culture; expression of CD73, CD90, CD105, and the absence of CD45, CD14, CD19, CD34; and differentiation toward osteogenic, adipogenic, and chondrogenic phenotypes.
MSCs from bone marrow are already the focus of numerous human clinical trials,31,32 and have shown enormous promise in preclinical studies to facilitate bone regeneration,33 promote tissue neovascularization,34–36 and reduce inflammation.37 There was a great deal of initial optimism that MSCs could differentiate into a wider variety of functional cell types (e.g., cardiac myocytes, neurons, ECs, etc.); however, after nearly 20 years of research, it is now clear that much of their therapeutic benefit occurs via trophic effects, i.e. through the secretion of numerous growth factors.37 There is widespread consensus that MSC trophic factors enhance angiogenesis and suppress inflammation, and as a result, many papers in the literature now refer to them as mesenchymal stromal cells, marrow stromal cells, or (more recently) “medicinal signaling cells”.30
It has more recently been demonstrated that MSCs can be isolated from other tissues, including fat, muscle, and numerous periodontal tissues.38–45 Though these sources of MSCs are all very different anatomically, a common microenvironmental feature is the high degree of vascularity. In a landmark paper, Crisan et al. identified MSCs in multiple human organs and tissues in vivo, including bone marrow, skeletal muscle, pancreas, placenta, white adipose tissue, and others (Fig. 2). In each of these tissues, MSCs reside next to capillaries larger than 10 μm in diameter and arterioles ranging from 10–100 μm in diameter.46 The conserved anatomic location led to the hypotheses that all MSCs are pericytes,47 and their proximity to the vasculature enables them to readily mobilize and travel in the bloodstream to sites of injury.48 Consistent with these concepts, MSCs home to sites of stroke49 and cancer,50 and produce paracrine effectors.51 On the other hand, there is also evidence suggesting that the pericytic phenotype and function is not inherent to all MSCs.52 Nonetheless, based upon the location of MSCs in the perivascular niche and the evidence of their interactions with ECs, it is reasonable to hypothesize that the perivascular niche is instructive for MSC fate.
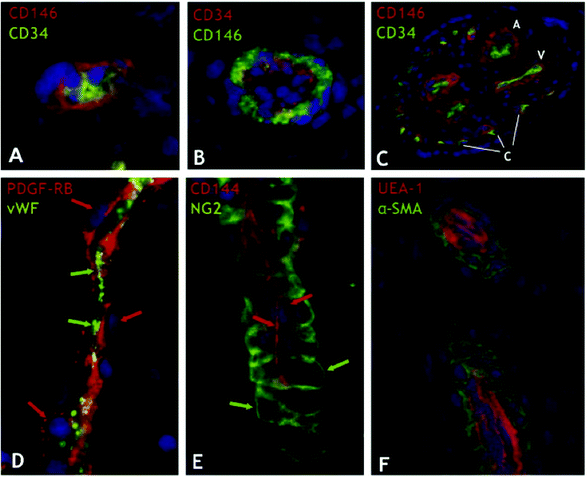 |
| Fig. 2 Perivascular niche of mesenchymal stem cells in human tissues. Human tissue sections from a wide range of organs were indirectly immunostained with antibodies to mural (CD146, PDGF-RB, NG2, and α-SMA) and endothelial cell markers (CD34, CD144, vWF, and UEA-1). (A) Myocardium: a capillary endothelial cell in transverse section marked by CD34 expression (green) is closely surrounded by a CD146+ pericyte (red) (×900). (B) Skeletal muscle: small vessel transverse section; CD146+ perivascular cells (green) surround CD34+ endothelial cells (red) (×400). (C) Placental villus: arterioles (A), venules (V), and capillaries (C) are all lined with CD34+ endothelial cells (green) surrounded by CD146+ perivascular cells (red) (×100). (D) Skeletal muscle: small vessel longitudinal section; PDGF-Rβ-positive perivascular cells (red; red arrows) surround vWF+ endothelial cells (green; green arrows) (×400). (E) Fetal pancreas: small vessel longitudinal section; NG2-positive perivascular cells (green; green arrows) surround CD144+ endothelial cells (red; red arrows) (×400). (F) Transverse and longitudinal sections of placental small blood vessels. Endothelial cells marked by the Ulex europaeus lectin (red) are surrounded by perivascular cells expressing α-SMA (green) (×400). Crisan et al. demonstrated that these perivascular cells were MSCs with multi-lineage potential. Adapted from Cell Stem Cell (Crisan et al.18) with permission from Elsevier. | |
The perivascular niche of hematopoietic stem cells
Hematopoietic stem cells (HSCs) are the self-renewing, multipotent progenitors that give rise to all mature blood cells. This multilineage potential is the underlying basis of bone marrow transplantation procedures, typically used to reconstitute the immune systems of patients with cancers of the blood or bone marrow (e.g., leukemia or multiple myeloma). The first bone marrow transplantation was performed in the late 1950s, and now tens of thousands of these procedures are done annually throughout the world. During embryonic development, the appearance of HSCs coincides with the appearance of blood vessels, both spatially and temporally, and then these cells establish hematopoiesis in the placenta, fetal liver, and spleen. HSCs primarily reside in the bone marrow in adult mammals, which becomes the major site of adult hematopoiesis.19
The niche of HSCs in adult mammals is perhaps the most widely studied of the adult stem cell niches, in part because of the fact that the existence of HSCs was first proposed more than 50 years ago to explain how bone marrow transplants could rescue mice that had received lethal doses of radiation.53 Many studies of the HSC niche have focused on the bone marrow endosteum, particularly the roles of osteoprogenitors and osteoblasts.19 Two studies in particular from 2003 showed that increasing the number of osteoblasts and the amount of trabecular bone in mouse models led to increased numbers of HSCs.54,55 However, bone is highly vascularized, and the marrow cavity contains vascular sinusoids that may also play critical roles in the regulation of HSC fate (Fig. 3).
 |
| Fig. 3 Perivascular niche of mesenchymal and hematopoietic stem cells. In the bone marrow, both MSCs and HSCs reside in the perivascular niche. The endothelial sinusoid is surrounded by MSCs identified as pericytes and a stromal subpopulation that can be further identified by their expression of nestin and/or leptin receptors. CAR cells are MSCs characterized by high expression of the chemokine CXCL12, a chemoattractant for CXCR4-expressing HSCs. Sympathetic neural fibers also connect with the niche and may provide negative proliferative signals. E-selectin is expressed exclusively on the endothelial cells of the niche and can bind HSCs expressing an appropriate ligand. ESC-1 is shown here as a candidate ligand, the binding of which to E-selectin is required for circulating HSCs to attach and roll on the sinusoidal endothelium before their firm attachment and egress. The attachment to E-selectin-expressing endothelium induces HSC proliferation, self-renewal and chemo- and radiosensitivity. HSCs also reside close to the bone endosteal surface (a more hypoxic environment) and may receive instructive cues from osteoblasts and osteoprogenitors. Reprinted by permission from Macmillan Publishers Ltd: Nature Medicine,127 copyright 2012. | |
The first evidence supporting an instructive role for the vasculature came from a study that used co-cultures of bone marrow sinusoidal ECs with human HSCs, demonstrating prolonged maintenance of the HSCs in culture.56,57 Kiel et al. identified a combination of signaling lymphocyte activation molecule (SLAM) receptors that could prospectively identify the locations of HSCs in tissue sections, and then used these markers to demonstrate that the majority of HSCs were associated with sinusoidal endothelium in spleen and bone marrow.58 This was a particularly important finding given the significant debate about whether the HSC niche was perivascular or endosteal.19 Consistent with a role for ECs in the HSC niche, another study showed that functional sinusoidal ECs in the bone marrow were essential for recovery of hematopoiesis after severe myelosuppression.59 Several other studies also support an instructive role for the vasculature in the bone marrow,60–63 and have identified candidate factors secreted by ECs that may regulate HSC self-renewal (discussed further below).
Nevertheless, given the many different cell types, adhesive surfaces, architectural features, and even mechanical properties present within the HSC niche, it seems likely that ECs are just one of many features that influence HSC fate. Other candidates include CD169+ macrophages64 and, perhaps somewhat unexpectedly, sympathetic neurons that innervate the bone marrow.65 The latter discovery offers a potential explanation for the chemotherapy-induced sensory neuropathy and hematopoietic dysfunction.
Perivascular niche in cancer
The angiogenic hypothesis, originally posed by Folkman, refers to the idea that tumor cells recruit host vasculature in order to enable a tumor to grow beyond the diffusion limit.66 This hypothesis has fueled extensive research over the past 30 years to better understand the signals used by tumor cells to recruit host vessels. Many new therapies target this aspect of cancer with the idea that inhibiting this process can starve a growing tumor and thereby potentially kill or at least prevent its further growth. However, as in the field of stem cell biology, the vasculature is increasingly viewed as an instructive niche for cancer cells, serving an important role beyond the delivery of oxygen and nutrients. One study in particular demonstrated that vessels within the brain provide a niche for self-renewing brain tumor cells with stem cell-like properties.67 Increasing the number of ECs or blood vessels in brain tumor xenografts expanded the number of these self-renewing and tumor initiating cells, while depleting them had the opposite effect and arrested tumor growth. In contrast to the restricted location of NSCs in the hippocampus and SVZ, the authors found that the brain tumor stem cells and associated vasculature were widely distributed throughout tumors and the brain.67
Other recent studies revealed that ECs directly influence cancer progression in unexpected ways inconsistent with the traditional tumor angiogenesis paradigm. For example, Franses et al. demonstrated that soluble secretions from quiescent ECs can mute the proliferative and invasive phenotype of lung and breast cancer cells both in vitro and in vivo. In addition, delivery of ECs in a scaffold adjacent to established xenograft tumors in mice actually suppressed growth of the tumors.68 This study suggests that ECs (and the factors they secrete) may actually suppress the growth of primary tumors.
In another study, Ghajar et al. showed that the perivascular niche provides a sort of “safe haven” for dormant disseminated breast cancer cells and controls the growth of secondary metastases.69 In this study using two different model organisms (mouse and zebrafish), the authors found dormant breast cancer cells on or near microvasculature in the lungs and/or bone marrow in vivo (Fig. 4). Using organotypic culture models composed of tissue-specific human microvascular networks, they then showed that stable microvasculature provided suppressive signals (specifically, thrombospondin-1) to maintain the tumor cells in their dormant state, whereas angiogenic vasculature (with sprouting endothelial tips) not only released the constraints that maintained dormancy but in fact accelerated cancer cell growth to form micrometastases. Sprouting EC-derived TGF-β1 and periostin were found to promote the growth of the dormant disseminated tumor cells.69 Other studies have also focused on the instructive role of ECs in the growth and spread of cancer cells,70,71 an important topic reviewed at length elsewhere.72 In the context of this review, the important point is that the perivascular niche is a critical regulator of both normal growth (i.e., for stem cells) and pathological growth (i.e., tumors).
 |
| Fig. 4 Dormant breast cancer cells reside in a perivascular niche following dissemination from the primary tumor. In an in vivo model of tumor cell dormancy, a weakly metastatic breast cancer cell line (T4-2, green) disseminated from the primary tumor and localized to perivascular niches (marked by CD31, red) in the (A) lungs, (B) bone marrow, and (C) brain. The cells are dormant as shown by the absence of the proliferation marker, Ki67. (D) In an organotypic in vitro model of the bone marrow microvasculature, the T4-2 cells remained dormant (Ki67 negative, denoted with white asterisks) when localized next to stable vasculature (further examples in panels ii–iv.), but were induced to a highly proliferative state when localized next to neovascular tips (denoted by T). These proliferative clusters have the potential to become new metastatic lesions. DNA was labeled with Hoechst 33342 (blue). Adapted by permission from Macmillan Publishers Ltd: Nature Cell Biology,69 copyright 2013. | |
Molecular regulation within the perivascular niche
The evidence reviewed thus far strongly suggests that the perivascular niche is the conserved anatomic location of many adult stem cell populations. However, the essential biomolecular and biophysical features of this niche, and the mechanisms by which they regulate stem cell self-renewal and differentiation, remain incompletely defined. It is critical to better define these essential features and mechanisms in order to engineer artificial stem cell niches using biomaterials and culture systems.
ECs secrete a wide range of important soluble proteins (collectively referred to as angiocrine factors), which have been implicated in the regulation of progenitor cells and tumor cells.72 Amongst these, nitric oxide (NO)73,74 and pigment epithelium-derived factor (PEDF)75,76 have been identified as important cues that regulate NSC self-renewal in the perivascular niche. Brain-derived neurotrophic factor (BDNF) from ECs also plays an important role in the NSC niche, as demonstrated by the finding that ECs co-cultured with explants of the adult SVZ from rats enhance neurogenesis via the release of BDNF.77 However, proper spatial and temporal regulation of BDNF is especially critical for neuronal differentiation, as its target appears to be the transit-amplifying progeny of NSCs rather than the NSCs themselves.21
Several other EC-derived cytokines have also been implicated in the perivascular niche, including IL-1, IL-3, IL-6, GCSF, and GM-CSF.72 In many cases, roles for these factors were first hypothesized based on known interactions of cancerous cells (i.e., leukemic cells) with the vasculature in the bone marrow. SDF1/CXCL12 was also recently demonstrated to be involved in triggering the migration of CXCR4-expressing neuroblasts from the subependymal region of the SVZ towards the microvasculature.78 Bone morphogenetic proteins secreted by ECs also regulate both NSCs79 and MSCs.80 Stem cell factor (SCF, also known as the c-Kit ligand) produced by ECs and other perivascular cells has been shown to maintain HSCs; when the gene encoding for SCF was deleted from ECs or a fraction of perivascular stromal cells in conditional knock-out mice, HSCs were depleted from the bone marrow.61
Adding to the complexity, insoluble cues in the form of cell–cell and cell–ECM interactions have also been implicated as instructive factors within the perivascular niche. Notch signaling pathways (cell–cell) in particular are important in the maintenance of both NSCs and HSCs, and ligands for these receptors (including Dll4 and Jagged) are expressed by ECs.21,60,72,81 Prior studies have shown that disruptions in Notch signaling impair the maintenance of HSCs both in vitro and in vivo,54,82 and it is plausible the ECs within the perivascular niche may be the predominant source of the Notch ligands. Another recent study demonstrated that E-selectin expression by sinusoidal ECs in the bone marrow regulates HSC dormancy (quiescence), self-renewal, and resistance to chemotherapies, and likely plays an important role in the homing of circulating HSCs to the bone marrow (Fig. 3).83 Although the reciprocal ligand expressed by the HSCs was not identified, the study noted the levels of E-selectin expression were markedly higher in sinusoids closer to the endosteal interface compared to the center of the bone marrow.
In the perivascular niches of both NSCs and MSCs, there is evidence that laminin deposited in the vascular basement membrane is an important instructive cue as well. Shen et al. showed that neural progenitors in the adult SVZ express the laminin receptor α6β1 integrin, and blocking this receptor alters their location in the perivascular niche and their proliferation in vivo.16 Similarly, a study from my laboratory showed that MSCs also express α6β1 integrin, and reduced expression of this integrin via RNA interference disrupted their association with laminin in an in vitro model of the perivascular niche and altered their ability to enhance angiogenic sprouting (Fig. 5).84
 |
| Fig. 5 An important integrin-mediated interaction in the perivascular niche. This cartoon illustrates the interaction of MSCs via their α6β1 integrin receptor with laminin in the vascular basement membrane. Disruption of this interaction blocks recruitment of MSCs to the perivascular niche and disrupts their ability to stimulate angiogenic sprouting by the ECs. Similar findings have been reported for NSCs in their perivascular niche.16 Adapted from Experimental Cell Research (Carrion et al.84) with permission from Elsevier. | |
Collectively, the papers cited here underscore the rapidly growing list of both soluble and insoluble angiocrine factors, and suggest that the mechanisms by which the perivascular niche regulates cell fate are complex and multivariate. However, the molecular genetic bias of the field of stem cell biology is reflected in this growing list of candidate molecules, while the possibility for biophysical control of cell fate within the perivascular niche remains largely ignored. Shear stress is a well-known modulator of EC physiology, and it is probable that blood flow can modulate the production of angiocrine factors by the ECs, even though blood flow rates in the capillary beds are slow compared to those in the arterioles and larger arteries.85 Example EC-secreted factors upregulated by shear stress include interleukin-1α (IL-1α), IL-8, and platelet derived growth factor (PDGF).86,87 ECs also upregulate their expression of numerous adhesion molecules and other receptors when exposed to shear stress,88 including the S1P receptor.89 Likewise, there is evidence that the intrinsic properties of the ECM regulate MSC fate decisions4 and HSC self-renewal90via processes that involve the ability of non-muscle myosin II to generate forces.91 Thus, despite differences in the elastic moduli (soft vs. hard) of the resulting tissues (brain vs. bone) that arise from different adult stem cell populations, the intrinsic mechanical properties of the vascular basement membrane within the conserved perivascular niche may in fact represent a common instructive signal that regulates self-renewal; this feature of the niche has yet to be examined critically. The contributions from other mechanical inputs within the niche (e.g., interstitial flow, applied loads, and forces from other cells) also need to be assessed as they too may have important roles.
Recreating the vascular niche in vitro and in vivo
There are many different strategies to dissect the perivascular niche and its abilities to regulate adult stem cell populations. Most insights have come from the use of model organisms, particularly rodent models, although there is increasing analysis of human tissue sections too. Studies examining endogenous niches in wild type and transgenic mice have relied on combinations of markers to distinguish stem cells from the vasculature and other stromal cell types.16–18 Vascularized tumor xenograft models have also been used to investigate the perivascular interactions of tumor stem cells.67 In the case of HSCs, conditional knock-out and transgenic mice have been used to explore the roles of specific angiocrine factors that maintain HSCs in vivo.60–62 On the other hand, a number of important insights regarding the perivascular niche of NSCs have come from studies examining the vocal control region of the forebrains of adult songbirds.21,25,92
The use of animal models is essential to better understand the critical instructive cues provided to MSCs, HSCs, and NSCs in their perivascular niche. However, animal models are not without their limitations, including the relatively high cost of generating and maintaining new colonies of transgenic animals and the possibility that subtle differences in human anatomy may complicate interpretations. As a complement to animal models, there is increased interest in applying tissue engineering principles to create appropriate model systems to study the regulation of stem cells in their perivascular niche. In the case of hematopoiesis specifically, tissue engineering approaches have been pursued for the past two decades in an effort to reconstitute hematopoiesis ex vivo.93 Simple in vitro models include co-cultures, for example of ECs with stem cells.15,60 More physiologically relevant co-cultures involve the formation of ECs in networks of microvasculature. There are now dozens of different in vitro models of vascularization,94–100 most of which have been employed to better understand the cues that drive angiogenic sprouting in both normal and tumorigenic models and have only more recently been explored as in vitro recreations of the perivascular niche.69,101,102 Many of these model systems are 3D co-cultures, in which ECs and other cell types are embedded within a natural protein-based ECM (i.e., collagen-I, fibrin, etc.) or a synthetic ECM analog (i.e., PEG103). In the model systems we employ in my laboratory, the microvasculature that forms contains hollow lumens with appropriate EC basolateral polarity and a collagen IV- and laminin 111-rich basement membrane.104,105 Similar model systems with the capability to perfuse the lumens, thereby mimicking blood flow, have also been described.94,95,97,106 Although much more complex than simple 2D co-cultures, these types of 3D multicellular in vitro models with anatomically correct microvasculature have the potential to yield new insights regarding the regulation of the perivascular stem cell niche in development, regeneration, and disease.
There are also a number of in vivo engineered tissue models potentially amenable to recreating and studying the perivascular niche in ectopic locations. One such model involves scaffold-based delivery of MSCs, which then recruit host vasculature and form new bone, and also recruit host HSCs, which occupy a functional marrow cavity and reconstitute hematopoiesis.107–109 Another model involves co-delivery of ECs (or endothelial progenitors) with MSCs (or some other multipotent stromal cell type) within a hydrogel precursor solution, which gels in situ entrapping the delivered cells and providing a suitable environment for vasculogenesis.110–115 A variation of this approach involves forming a pre-vascularized tissue construct in vitro first, followed by subsequent implantation into an animal where the pre-formed vessels can rapidly inosculate with host vessels.116,117 Although these model systems have not been fully exploited for the purposes of recreating the perivascular niche, they offer a potentially powerful tool to systematically investigate how niche-specific cues regulate stem cell self-renewal and lineage specification and thereby complement the use of transgenic mice.
Finally, combining advances in biomaterials science with increased knowledge of the signals present within the perivascular niche provides an opportunity to create artificial stem cell niches that can direct cell fate in vivo. An example of this approach was recently described by Conway and Schaffer.118 In this study, recombinant versions of two known neurogenic signals (Sonic hedgehog and ephrin-B2) were conjugated to 800 kDa hyaluronic acid to create multivalent, highly active ligands that mimic signals founds in native neurogenic niches. Delivery of these materials into non-neurogenic regions of the brain yielded the formation of new neurons, and partially rescued the neurogenic potential of geriatric rodent brains towards the levels of young animals. Co-delivery of a stromal cell-derived factor-1α with the multivalent biomaterial ligands further increased neurogenesis to levels that may be therapeutic. This approach offers the potential to replace neurons lost due to degenerative diseases or injuries without the need for cell transplantation, an exciting possibility for the field of regenerative medicine. In addition, as new factors that influence cell fate decisions (including those from the perivascular niche) are identified, they could be similarly conjugated to polymers and used to stimulate developmental programs and tissue regeneration.
Implications and future directions
From the discussion of key papers presented here in this review, it is clear that the perivascular niche provides important instructive cues that regulate stem cell self-renewal and multi-lineage specification. Understanding the precise spatial and temporal presentation of these cues is a significant challenge that will require substantial time, effort, and resources, and the use of new tools and innovative strategies. However, the potential payoff of such an improved and mechanistic understanding is also impressive, and there are far-reaching implications for investigators working in the fields of biomaterials and regenerative medicine. For example, key angiocrine soluble cues will undoubtedly continue to be identified from studies of endogenous and recreated perivascular niches, along with insoluble and perhaps physical cues intrinsic to the perivascular microenvironment. Biomaterial platforms capable of controlled release of multiple factors with distinct kinetics119 may play key roles in better defining niche-specific stem cell regulation, and be applied to exploit findings for therapeutic applications in regenerative medicine. As highlighted in the previous section, tissue engineering strategies that recreate the perivascular niche both in vitro and in vivo have already lead to improved understanding, and will undoubtedly continue to provide new fundamental insights.
Blank slate biomaterials that present inert backgrounds to cells and can be covalently functionalized to present niche-specific adhesive cues (e.g., PEG-based hydrogels) will also be essential tools to effectively recapitulate features of the perivascular niche.11,120 Such materials are already leading to new insights regarding the instructive roles of stem cell niches, both for adult stem cells121 and embryonic stem cells.122 Furthermore, because the number of potential adhesive signals is so large, combinatorial biomaterial approaches have been developed to examine hundreds and perhaps thousands of different ligands and/or combinations for their potential to control the fate of clonal populations of stem cells.121,123 Incorporating diffusible gradients of soluble morphogens via microfluidic networks into engineered microenvironments is another important approach.124 Material platforms that allow the decoupling of multiple instructive signals in order to pinpoint the roles of each one individually would undoubtedly be useful tools in these efforts as well, but it is clear that this remains a challenge as materials once considered “bulletproof” with respect to this decoupling have recently been questioned.125 When cells are entrapped in 3D materials, the coupling of adhesive, mechanical, and diffusive signals becomes potentially even more complex.126 Nevertheless, I firmly believe that the bioengineering community will play a significant role in dissecting the multiple instructive cues present within perivascular niches, and develop tools and approaches to enable these cues to be exploited for therapeutic purposes.
Acknowledgements
The author gratefully acknowledges financial support from the National Heart, Lung, and Blood Institute of the National Institutes of Health under award numbers R01HL085339 and R01HL118259.
References
- R. Schofield, The relationship between the spleen colony-forming cell and the haemopoietic stem cell, Blood Cells, 1978, 4(1–2), 7 CAS
.
- D. E. Discher, D. J. Mooney and P. W. Zandstra, Growth factors, matrices, and forces combine and control stem cells, Science, 2009, 324(5935), 1673 CrossRef CAS PubMed
.
- K. A. Moore and I. R. Lemischka, Stem cells and their niches, Science, 2006, 311(5769), 1880 CrossRef CAS PubMed
.
- A. J. Engler, S. Sen, H. L. Sweeney and D. E. Discher, Matrix Elasticity Directs Stem Cell Lineage Specification, Cell, 2006, 126(4), 677 CrossRef CAS PubMed
.
- K. Saha,
et al., Substrate modulus directs neural stem cell behavior, Biophys. J., 2008, 95(9), 4426 CrossRef CAS PubMed
.
- P. M. Gilbert,
et al., Substrate elasticity regulates skeletal muscle stem cell self-renewal in culture, Science, 2010, 329(5995), 1078 CrossRef CAS PubMed
.
- D. T. Scadden, The stem-cell niche as an entity of action, Nature, 2006, 441(7097), 1075 CrossRef CAS PubMed
.
- E. Fuchs, T. Tumbar and G. Guasch, Socializing with the neighbors: stem cells and their niche, Cell, 2004, 116(6), 769 CrossRef CAS
.
- C. Bordignon, Stem-cell therapies for blood diseases, Nature, 2006, 441(7097), 1100 CrossRef CAS PubMed
.
- N. S. Hwang, S. Varghese and J. Elisseeff, Controlled differentiation of stem cells, Adv. Drug Delivery Rev., 2008, 60(2), 199 CrossRef CAS PubMed
.
- M. P. Lutolf, P. M. Gilbert and H. M. Blau, Designing materials to direct stem-cell fate, Nature, 2009, 462(7272), 433 CrossRef CAS PubMed
.
- L. S. Ferreira,
et al., Bioactive hydrogel scaffolds for controllable vascular differentiation of human embryonic stem cells, Biomaterials, 2007, 28(17), 2706 CrossRef CAS PubMed
.
- T. Vazin and D. V. Schaffer, Engineering strategies to emulate the stem cell niche, Trends Biotechnol., 2010, 28(3), 117 CrossRef CAS PubMed
.
- C. M. Kolf, E. Cho and R. S. Tuan, Mesenchymal stromal cells. Biology of adult mesenchymal stem cells: regulation of niche, self-renewal and differentiation, Arthritis Res. Ther., 2007, 9(1), 204 Search PubMed
.
- Q. Shen,
et al., Endothelial cells stimulate self-renewal and expand neurogenesis of neural stem cells, Science, 2004, 304(5675), 1338 CrossRef CAS PubMed
.
- Q. Shen,
et al., Adult SVZ stem cells lie in a vascular niche: a quantitative analysis of niche cell-cell interactions, Cell Stem Cell, 2008, 3(3), 289 CrossRef CAS PubMed
.
- M. Tavazoie,
et al., A specialized vascular niche for adult neural stem cells, Cell Stem Cell, 2008, 3(3), 279 CrossRef CAS PubMed
.
- M. Crisan,
et al., A perivascular origin for mesenchymal stem cells in multiple human organs, Cell Stem Cell, 2008, 3(3), 301 CrossRef CAS PubMed
.
- M. J. Kiel and S. J. Morrison, Uncertainty in the niches that maintain haematopoietic stem cells, Nat. Rev. Immunol., 2008, 8(4), 290 CrossRef CAS PubMed
.
- F. H. Gage, Mammalian neural stem cells, Science, 2000, 287(5457), 1433 CrossRef CAS
.
- S. A. Goldman and Z. Chen, Perivascular instruction of cell genesis and fate in the adult brain, Nat. Neurosci., 2011, 14(11), 1382 CrossRef CAS PubMed
.
- S. Temple, Division and differentiation of isolated CNS blast cells in microculture, Nature, 1989, 340(6233), 471 CrossRef CAS PubMed
.
- F. Doetsch, A niche for adult neural stem cells, Curr. Opin. Genet. Dev., 2003, 13(5), 543 CrossRef CAS PubMed
.
- T. D. Palmer, A. R. Willhoite and F. H. Gage, Vascular niche for adult hippocampal neurogenesis, J. Comp. Neurol., 2000, 425(4), 479 CrossRef CAS
.
- A. Louissaint Jr., S. Rao, C. Leventhal and S. A. Goldman, Coordinated interaction of neurogenesis and angiogenesis in the adult songbird brain, Neuron, 2002, 34(6), 945 CrossRef
.
- P. Codega,
et al., Prospective identification and purification of quiescent adult neural stem cells from their in vivo niche, Neuron, 2014, 82(3), 545 CrossRef CAS PubMed
.
- A. J. Friedenstein, K. V. Petrakova, A. I. Kurolesova and G. P. Frolova, Heterotopic of bone marrow. Analysis of precursor cells for osteogenic and hematopoietic tissues, Transplantation, 1968, 6(2), 230 CrossRef CAS PubMed
.
- A. J. Friedenstein,
et al., Origin of bone marrow stromal mechanocytes in radiochimeras and heterotopic transplants, Exp. Hematol., 1978, 6(5), 440 CAS
.
- M. F. Pittenger,
et al., Multilineage potential of adult human mesenchymal stem cells, Science, 1999, 284(5411), 143 CrossRef CAS
.
- A. I. Caplan, What's in a name?, Tissue Eng., Part A, 2010, 16(8), 2415 CrossRef PubMed
.
- A. Giordano, U. Galderisi and I. R. Marino, From the laboratory bench to the patient's bedside: an update on clinical trials with mesenchymal stem cells, J. Cell Physiol., 2007, 211(1), 27 CrossRef CAS PubMed
.
- J. Wagner, T. Kean, R. Young, J. E. Dennis and A. I. Caplan, Optimizing mesenchymal stem cell-based therapeutics, Curr. Opin. Biotechnol., 2009, 20(5), 531 CrossRef CAS PubMed
.
- C. A. Simmons, E. Alsberg, S. Hsiong, W. J. Kim and D. J. Mooney, Dual growth factor delivery and controlled scaffold degradation enhance in vivo bone formation by transplanted bone marrow stromal cells, Bone, 2004, 35(2), 562 CrossRef CAS PubMed
.
- N. Nagaya,
et al., Transplantation of mesenchymal stem cells improves cardiac function in a rat model of dilated cardiomyopathy, Circulation, 2005, 112(8), 1128 CrossRef PubMed
.
- G. V. Silva,
et al., Mesenchymal stem cells differentiate into an endothelial phenotype, enhance vascular density, and improve heart function in a canine chronic ischemia model, Circulation, 2005, 111(2), 150 CrossRef CAS PubMed
.
- T. Kinnaird,
et al., Local delivery of marrow-derived stromal cells augments collateral perfusion through paracrine mechanisms, Circulation, 2004, 109(12), 1543 CrossRef CAS PubMed
.
- A. I. Caplan, Adult mesenchymal stem cells for tissue engineering versus regenerative medicine, J. Cell Physiol., 2007, 213(2), 341 CrossRef CAS PubMed
.
- B. M. Seo,
et al., Investigation of multipotent postnatal stem cells from human periodontal ligament, Lancet, 2004, 364(9429), 149 CrossRef CAS
.
- P. A. Zuk,
et al., Multilineage cells from human adipose tissue: implications for cell-based therapies, Tissue Eng., 2001, 7(2), 211 CrossRef CAS PubMed
.
- S. O. Akintoye,
et al., Skeletal site-specific characterization of orofacial and iliac crest human bone marrow stromal cells in same individuals, Bone, 2006, 38(6), 758 CrossRef CAS PubMed
.
- S. Gronthos, M. Mankani, J. Brahim, P. G. Robey and S. Shi, Postnatal human dental pulp stem cells (DPSCs) in vitro and in vivo, Proc. Natl. Acad. Sci. U. S. A., 2000, 97(25), 13625 CrossRef CAS PubMed
.
- M. Miura,
et al., SHED: stem cells from human exfoliated deciduous teeth, Proc. Natl. Acad. Sci. U. S. A., 2003, 100(10), 5807 CrossRef CAS PubMed
.
- W. M. Jackson, L. J. Nesti and R. S. Tuan, Potential therapeutic applications of muscle-derived mesenchymal stem and progenitor cells, Expert Opin. Biol. Ther., 2010, 10(4), 505 CrossRef CAS PubMed
.
- Q. Z. Zhang, A. L. Nguyen, W. H. Yu and A. D. Le, Human oral mucosa and gingiva: a unique reservoir for mesenchymal stem cells, J. Dental Res., 2012, 91(11), 1011 CrossRef CAS PubMed
.
- S. Mason, S. A. Tarle, W. Osibin, Y. Kinfu and D. Kaigler, Standardization and Safety of Alveolar Bone-derived Stem Cell Isolation, J. Dent. Res., 2014, 93(1), 55 CrossRef CAS PubMed
.
- M. Crisan,
et al., A Perivascular Origin for Mesenchymal Stem Cells in Multiple Human Organs, Cell Stem Cell, 2008, 3(3), 301 CrossRef CAS PubMed
.
- A. I. Caplan, All MSCs are pericytes?, Cell Stem Cell, 2008, 3(3), 229 CrossRef CAS PubMed
.
- L. da Silva Meirelles, A. M. Fontes, D. T. Covas and A. I. Caplan, Mechanisms involved in the therapeutic properties of mesenchymal stem cells, Cytokine Growth Factor Rev., 2009, 20(5–6), 419 CrossRef PubMed
.
- E. Kokovay, L. Li and L. A. Cunningham, Angiogenic recruitment of pericytes from bone marrow after stroke, J. Cereb. Blood Flow Metab., 2005, 26(4), 545 CrossRef PubMed
.
- B. M. Beckermann,
et al., VEGF expression by mesenchymal stem cells contributes to angiogenesis in pancreatic carcinoma, Br. J. Cancer, 2008, 99(4), 622 CrossRef CAS PubMed
.
- J. Doorn, G. Moll, K. Le Blanc, C. van Blitterswijk and J. de Boer, Therapeutic applications of mesenchymal stromal cells: paracrine effects and potential improvements, Tissue Eng., Part B, 2011, 18(2), 101 CrossRef PubMed
.
- A. Blocki,
et al., Not all MSCs can act as pericytes: functional in vitro assays to distinguish pericytes from other mesenchymal stem cells in angiogenesis, Stem Cells Dev., 2013, 22(17), 2347 CrossRef CAS PubMed
.
- I. L. Weissman and J. A. Shizuru, The origins of the identification and isolation of hematopoietic stem cells, and their capability to induce donor-specific transplantation tolerance and treat autoimmune diseases, Blood, 2008, 112(9), 3543 CrossRef CAS PubMed
.
- L. M. Calvi,
et al., Osteoblastic cells regulate the haematopoietic stem cell niche, Nature, 2003, 425(6960), 841 CrossRef CAS PubMed
.
- J. Zhang,
et al., Identification of the haematopoietic stem cell niche and control of the niche size, Nature, 2003, 425(6960), 836 CrossRef CAS PubMed
.
- S. Rafii,
et al., Human bone marrow microvascular endothelial cells support long-term proliferation and differentiation of myeloid and megakaryocytic progenitors, Blood, 1995, 86(9), 3353 CAS
.
- S. Rafii,
et al., Isolation and characterization of human bone marrow microvascular endothelial cells: hematopoietic progenitor cell adhesion, Blood, 1994, 84(1), 10 CAS
.
- M. J. Kiel, O. H. Yilmaz, T. Iwashita, C. Terhorst and S. J. Morrison, SLAM family receptors distinguish hematopoietic stem and progenitor cells and reveal endothelial niches for stem cells, Cell, 2005, 121(7), 1109 CrossRef CAS PubMed
.
- A. T. Hooper,
et al., Engraftment and reconstitution of hematopoiesis is dependent on VEGFR2-mediated regeneration of sinusoidal endothelial cells, Cell Stem Cell, 2009, 4(3), 263 CrossRef CAS PubMed
.
- J. M. Butler,
et al., Endothelial cells are essential for the self-renewal and repopulation of Notch-dependent hematopoietic stem cells, Cell Stem Cell, 2010, 6(3), 251 CrossRef CAS PubMed
.
- L. Ding, T. L. Saunders, G. Enikolopov and S. J. Morrison, Endothelial and perivascular cells maintain haematopoietic stem cells, Nature, 2012, 481(7382), 457 CrossRef CAS PubMed
.
- H. Kobayashi,
et al., Angiocrine factors from Akt-activated endothelial cells balance self-renewal and differentiation of haematopoietic stem cells, Nat. Cell Biol., 2010, 12(11), 1046 CrossRef CAS PubMed
.
- Y. Kunisaki,
et al., Arteriolar niches maintain haematopoietic stem cell quiescence, Nature, 2013, 502(7473), 637 CrossRef CAS PubMed
.
- A. Chow,
et al., CD169(+) macrophages provide a niche promoting erythropoiesis under homeostasis and stress, Nat. Med., 2013, 19(4), 429 CrossRef CAS PubMed
.
- D. Lucas,
et al., Chemotherapy-induced bone marrow nerve injury impairs hematopoietic regeneration, Nat. Med., 2013, 19(6), 695 CrossRef CAS PubMed
.
- J. Folkman, Angiogenesis in cancer, vascular, rheumatoid and other disease, Nat. Med., 1995, 1(1), 27 CrossRef CAS PubMed
.
- C. Calabrese,
et al., A perivascular niche for brain tumor stem cells, Cancer Cell, 2007, 11(1), 69 CrossRef CAS PubMed
.
- J. W. Franses, A. B. Baker, V. C. Chitalia and E. R. Edelman, Stromal endothelial cells directly influence cancer progression, Sci. Transl. Med., 2011, 3(66), 66ra5 Search PubMed
.
- C. M. Ghajar,
et al., The perivascular niche regulates breast tumour dormancy, Nat. Cell Biol., 2013, 15(7), 807 CrossRef CAS PubMed
.
- S. Bandyopadhyay,
et al., Interaction of KAI1 on tumor cells with DARC on vascular endothelium leads to metastasis suppression, Nat. Med., 2006, 12(8), 933 CrossRef CAS PubMed
.
- S. Indraccolo,
et al., Cross-talk between tumor and endothelial cells involving the Notch3-Dll4 interaction marks escape from tumor dormancy, Cancer Res., 2009, 69(4), 1314 CrossRef CAS PubMed
.
- J. M. Butler, H. Kobayashi and S. Rafii, Instructive role of the vascular niche in promoting tumour growth and tissue repair by angiocrine factors, Nat. Rev. Cancer, 2010, 10(2), 138 CrossRef CAS PubMed
.
- M. A. Packer,
et al., Nitric oxide negatively regulates mammalian adult neurogenesis, Proc. Natl. Acad. Sci. U. S. A., 2003, 100(16), 9566 CrossRef CAS PubMed
.
- B. P. Carreira,
et al., Nitric oxide stimulates the proliferation of neural stem cells bypassing the epidermal growth factor receptor, Stem Cells, 2010, 28(7), 1219 CAS
.
- C. Ramirez-Castillejo,
et al., Pigment epithelium-derived factor is a niche signal for neural stem cell renewal, Nat. Neurosci., 2006, 9(3), 331 CrossRef CAS PubMed
.
- C. Andreu-Agullo, J. M. Morante-Redolat, A. C. Delgado and I. Farinas, Vascular niche factor PEDF modulates Notch-dependent stemness in the adult subependymal zone, Nat. Neurosci., 2009, 12(12), 1514 CrossRef CAS PubMed
.
- C. Leventhal, S. Rafii, D. Rafii, A. Shahar and S. A. Goldman, Endothelial trophic support of neuronal production and recruitment from the adult mammalian subependyma, Mol. Cell. Neurosci., 1999, 13(6), 450 CrossRef CAS PubMed
.
- E. Kokovay,
et al., Adult SVZ lineage cells home to and leave the vascular niche via differential responses to SDF1/CXCR4 signaling, Cell Stem Cell, 2010, 7(2), 163 CrossRef CAS PubMed
.
- C. Mathieu,
et al., Endothelial cell-derived bone morphogenetic proteins control proliferation of neural stem/progenitor cells, Mol. Cell. Neurosci., 2008, 38(4), 569 CrossRef CAS PubMed
.
- D. Kaigler,
et al., Endothelial cell modulation of bone marrow stromal cell osteogenic potential, FASEB J., 2005, 19(1), 665 CAS
.
- M. G. Poulos,
et al., Endothelial Jagged-1 is necessary for homeostatic and regenerative hematopoiesis, Cell Rep., 2013, 4(5), 1022 CrossRef CAS PubMed
.
- B. K. Hadland,
et al., A requirement for Notch1 distinguishes 2 phases of definitive hematopoiesis during development, Blood, 2004, 104(10), 3097 CrossRef CAS PubMed
.
- I. G. Winkler,
et al., Vascular niche E-selectin regulates hematopoietic stem cell dormancy, self renewal and chemoresistance, Nat. Med., 2012, 18(11), 1651 CrossRef CAS PubMed
.
- B. Carrion, Y. P. Kong, D. Kaigler and A. J. Putnam, Bone marrow-derived mesenchymal stem cells enhance angiogenesis via their alpha6beta1 integrin receptor, Exp. Cell Res., 2013, 319(19), 2964 CrossRef CAS PubMed
.
- J. Zhou, Y. S. Li and S. Chien, Shear Stress-Initiated Signaling and Its Regulation of Endothelial Function, Arterioscler. Thromb. Vasc. Biol., 2014 DOI:10.1161/ATVBAHA.114.303422
.
- A. Dardik, A. Yamashita, F. Aziz, H. Asada and B. E. Sumpio, Shear stress-stimulated endothelial cells induce smooth muscle cell chemotaxis via platelet-derived growth factor-BB and interleukin-1alpha, J. Vasc. Surg., 2005, 41(2), 321 CrossRef PubMed
.
- K. Urschel, I. Cicha, W. G. Daniel and C. D. Garlichs, Shear stress patterns affect the secreted chemokine profile in endothelial cells, Clin. Hemorheol. Microcirc., 2012, 50(1–2), 143 Search PubMed
.
- H. J. Lee, N. Li, S. M. Evans, M. F. Diaz and P. L. Wenzel, Biomechanical force in blood development: extrinsic physical cues drive pro-hematopoietic signaling, Differentiation, 2013, 86(3), 92 CrossRef CAS PubMed
.
- B. Jung,
et al., Flow-regulated endothelial S1P receptor-1 signaling sustains vascular development, Dev. Cell, 2012, 23(3), 600 CrossRef CAS PubMed
.
- J. Holst,
et al., Substrate elasticity provides mechanical signals for the expansion of hemopoietic stem and progenitor cells, Nat. Biotechnol., 2010, 28(10), 1123 CrossRef CAS PubMed
.
- J. W. Shin,
et al., Contractile forces sustain and polarize hematopoiesis from stem and progenitor cells, Cell Stem Cell, 2014, 14(1), 81 CrossRef CAS PubMed
.
- S. A. Goldman and F. Nottebohm, Neuronal production, migration, and differentiation in a vocal control nucleus of the adult female canary brain, Proc. Natl. Acad. Sci. U. S. A., 1983, 80(8), 2390 CrossRef CAS
.
- M. R. Koller and B. O. Palsson, Review: tissue engineering: reconstitution of human hematopoiesis ex vivo, Biotechnol. Bioeng., 1993, 42(8), 909 CrossRef CAS PubMed
.
- Y. Zheng,
et al., In vitro microvessels for the study of angiogenesis and thrombosis, Proc. Natl. Acad. Sci. U. S. A., 2012, 109(24), 9342 CrossRef CAS PubMed
.
- J. H. Yeon, H. R. Ryu, M. Chung, Q. P. Hu and N. L. Jeon, In vitro formation and characterization of a perfusable three-dimensional tubular capillary network in microfluidic devices, Lab Chip, 2012, 12(16), 2815 RSC
.
- V. Nehls and D. Drenckhahn, A microcarrier-based cocultivation system for the investigation of factors and cells involved in angiogenesis in three-dimensional fibrin matrices in vitro, Histochem. Cell Biol., 1995, 104(6), 459 CrossRef CAS
.
- J. S. Miller,
et al., Rapid casting of patterned vascular networks for perfusable engineered three-dimensional tissues, Nat. Mater., 2012, 11(9), 768 CrossRef CAS PubMed
.
- A. N. Stratman, K. M. Malotte, R. D. Mahan, M. J. Davis and G. E. Davis, Pericyte recruitment during vasculogenic tube assembly stimulates endothelial basement membrane matrix formation, Blood, 2009, 114(24), 5091 CrossRef CAS PubMed
.
- K. H. Wong, J. M. Chan, R. D. Kamm and J. Tien, Microfluidic models of vascular functions, Annu. Rev. Biomed. Eng., 2012, 14, 205 CrossRef CAS PubMed
.
- D. Hanjaya-Putra,
et al., Controlled activation of morphogenesis to generate a functional human microvasculature in a synthetic matrix, Blood, 2011, 118(3), 804 CrossRef CAS PubMed
.
- B. Carrion,
et al., Recreating the perivascular niche ex vivo using a microfluidic approach, Biotechnol. Bioeng., 2010, 107(6), 1020 CrossRef CAS PubMed
.
- D. W. Infanger, M. E. Lynch and C. Fischbach, Engineered culture models for studies of tumor-microenvironment interactions, Annu. Rev. Biomed. Eng., 2013, 15, 29 CrossRef CAS PubMed
.
- R. K. Singh, D. Seliktar and A. J. Putnam, Capillary morphogenesis in PEG-collagen hydrogels, Biomaterials, 2013, 34(37), 9331 CrossRef CAS PubMed
.
- C. M. Ghajar, K. S. Blevins, C. C. Hughes, S. C. George and A. J. Putnam, Mesenchymal Stem Cells Enhance Angiogenesis in Mechanically Viable Prevascularized Tissues via Early Matrix Metalloproteinase Upregulation, Tissue Eng., 2006, 12(10), 2875 CrossRef CAS PubMed
.
- C. M. Ghajar,
et al., Mesenchymal cells stimulate capillary morphogenesis via distinct proteolytic mechanisms, Exp. Cell Res., 2010, 316(5), 813 CrossRef CAS PubMed
.
- J. S. Jeon,
et al., Generation of 3D functional microvascular networks with human mesenchymal stem cells in microfluidic systems, Integr. Biol., 2014, 6(5), 555 RSC
.
- J. Song,
et al., An in vivo model to study and manipulate the hematopoietic stem cell niche, Blood, 2010, 115(13), 2592 CrossRef CAS PubMed
.
- J. Lee,
et al., Implantable microenvironments to attract hematopoietic stem/cancer cells, Proc. Natl. Acad. Sci. U. S. A., 2012, 109(48), 19638 CrossRef CAS PubMed
.
- C. Scotti,
et al., Engineering of a functional bone organ through endochondral ossification, Proc. Natl. Acad. Sci. U. S. A., 2013, 110(10), 3997 CrossRef CAS PubMed
.
- P. Allen, J. Melero-Martin and J. Bischoff, Type I collagen, fibrin and PuraMatrix matrices provide permissive environments for human endothelial and mesenchymal progenitor cells to form neovascular networks, J. Tissue Eng. Regen. Med., 2011, 5(4), e74 CrossRef CAS PubMed
.
- J. M. Melero-Martin,
et al., Engineering Robust and Functional Vascular Networks In Vivo With Human Adult and Cord Blood–Derived Progenitor Cells, Circ. Res., 2008, 103, 128 CrossRef PubMed
.
- N. Koike,
et al., Tissue engineering: creation of long-lasting blood vessels, Nature, 2004, 428(6979), 138 CrossRef CAS PubMed
.
- J. S. Schechner,
et al., In vivo formation of complex microvessels lined by human endothelial cells in an immunodeficient mouse, Proc. Natl. Acad. Sci. U. S. A., 2000, 97(16), 9191 CrossRef CAS PubMed
.
- S. J. Grainger, B. Carrion, J. Ceccarelli and A. J. Putnam, Stromal cell identity influences the in vivo functionality of engineered capillary networks formed by co-delivery of endothelial cells and stromal cells, Tissue Eng., Part A, 2013, 19(9–10), 1209 CrossRef CAS PubMed
.
- E. Kniazeva, S. Kachgal and A. J. Putnam, Effects of extracellular matrix density and mesenchymal stem cells on neovascularization in vivo, Tissue Eng., Part A, 2011, 17(7–8), 905 CrossRef CAS PubMed
.
- X. Chen,
et al., Prevascularization of a fibrin-based tissue construct accelerates the formation of functional anastomosis with host vasculature, Tissue Eng., Part A, 2009, 15(6), 1363 CrossRef CAS PubMed
.
- X. Chen,
et al., Rapid anastomosis of endothelial progenitor cell-derived vessels with host vasculature is promoted by a high density of cotransplanted fibroblasts, Tissue Eng., Part A, 2010, 16(2), 585 CrossRef CAS PubMed
.
- A. Conway and D. V. Schaffer, Biomaterial
microenvironments to support the generation of new neurons in the adult brain, Stem Cells, 2014, 32(5), 1220 CrossRef CAS PubMed
.
- T. P. Richardson, M. C. Peters, A. B. Ennett and D. J. Mooney, Polymeric system for dual growth factor delivery, Nat. Biotechnol., 2001, 19(11), 1029 CrossRef CAS PubMed
.
- M. P. Lutolf and J. A. Hubbell, Synthetic biomaterials as instructive extracellular microenvironments for morphogenesis in tissue engineering, Nat. Biotechnol., 2005, 23(1), 47 CrossRef CAS PubMed
.
- S. Gobaa,
et al., Artificial niche microarrays for probing single stem cell fate in high throughput, Nat. Methods, 2011, 8(11), 949 CrossRef CAS PubMed
.
- S. T. Lee,
et al., Engineering integrin signaling for promoting embryonic stem cell self-renewal in a precisely defined niche, Biomaterials, 2010, 31(6), 1219 CrossRef CAS PubMed
.
- Y. Mei,
et al., Combinatorial development of biomaterials for clonal growth of human pluripotent stem cells, Nat. Mater., 2010, 9(9), 768 CrossRef CAS PubMed
.
- S. Kobel and M. P. Lutolf, Biomaterials meet microfluidics: building the next generation of artificial niches, Curr. Opin. Biotechnol., 2011, 22(5), 690 CrossRef CAS PubMed
.
- B. Trappmann,
et al., Extracellular-matrix tethering regulates stem-cell fate, Nat. Mater., 2012, 11(7), 642 CrossRef CAS PubMed
.
- C. M. Ghajar,
et al., The effect of matrix density on the regulation of 3-D capillary morphogenesis, Biophys. J., 2008, 94(5), 1930 CrossRef CAS PubMed
.
- M. A. Moore, Waking up HSCs: a new role for E-selectin, Nat. Med., 2012, 18(11), 1613 CrossRef CAS PubMed
.
|
This journal is © The Royal Society of Chemistry 2014 |
Click here to see how this site uses Cookies. View our privacy policy here.