DOI:
10.1039/C4BM00304G
(Paper)
Biomater. Sci., 2014,
2, 1706-1714
The effect of endothelial cells on hESC-derived pancreatic progenitors in a 3D environment†
Received
17th August 2014
, Accepted 26th August 2014
First published on 15th September 2014
Abstract
Generating transplantable β-like-cells from human embryonic stem cells (hESC) could serve as an ideal cell-based therapy for treatment of type 1 diabetes, which is characterized by the destruction of insulin-secreting pancreatic β-cells. There are several protocols for differentiating hESCs into pancreatic or endocrine precursors. However, so far, production of mature, functional β-like-cells has been achieved mainly by transplanting hESC derived pancreatic progenitors (PPs) and allowing several months for maturation to occur in vivo. One approach, believed to have potential in promoting differentiation into β-like-cells prior to transplantation, is culturing PPs alongside blood vessels. Endothelium and blood vessels have been shown to direct pancreatic development during embryogenesis and also induce endocrine differentiation in vitro. Here we designed a three-dimensional (3D) construct utilizing highly porous polymeric scaffolds that mimic natural conditions and provide cells with mechanical support, and used it in the differentiation protocol. Clusters of hESC derived pancreatic precursor cells were embedded within the scaffolds along with human endothelial cells (ECs) and fibroblasts forming vessel-like networks. Culturing these clusters with ECs for one week significantly increased the population of PPs, characterized by co-expression of the pancreatic markers Pdx1 and Nkx6.1 and also highly induced Ngn3 expression which indicates commitment to endocrine fate. The presence of fibroblasts, however, reduced this cell population. Three months upon implantation of constructs containing clusters and ECs or clusters alone, implanted mice retained normal blood glucose levels after treatment with STZ, while un-implanted mice became diabetic. These findings may lay the foundation for creating an optimal tissue-construct that will support PPs’ maturation in vitro and enhance graft function upon implantation.
Introduction
Islet transplantation has attracted much attention in the last decade since its introduction as an effective, minimally invasive method of restoring glycemic control in type 1 diabetics.1 However, this approach is currently hampered by low survival rates of transplanted islets which increase the number of donors required per transplantation, a very limiting factor given the severe shortage in organ donors. Furthermore, most islet recipients experience a reoccurrence of diabetes several years post-transplantation and return to relying on exogenous insulin intake.2 Consequently, tremendous efforts are invested in finding an alternative source for islet β-cells that could be mass manufactured and transplanted repeatedly, thus serving as a widespread cell therapy for type 1 diabetes.3 Human embryonic stem cells (hESCs) have emerged as a leading candidate for this target due to their unlimited capacity to proliferate and differentiation potential.4 Extensive research has been conducted in recent years in order to develop an effective protocol to direct hESC differentiation into functional β-cells. To date, several groups have succeeded in generation of pancreatic progenitors (PPs),5–9 but final maturation into β-like-cells has been achieved after a lengthy in vivo implantation period.7,9
One of the central reasons for the low survival rate of transplanted islets is the lack of sufficient vasculature shortly after transplantation. Islets are naturally highly dependent on their extensive intravascular system which is severed during isolation, leaving the islets completely reliant on the host vasculature until revascularization is attained.10 During this time islets are extremely susceptible to ischemia and increased mortality.11 Furthermore, beyond their role in blood supply, studies have shown that blood vessels and the endothelium are key players in the promotion of growth and differentiation of embryonic pancreas into an endocrine fate.12–14 This effect was also reported to be seen in vitro, even in the absence of blood flow.15 Hence, we hypothesized that culturing hESC derived pancreatic cells in vitro, in a 3D environment and a vascular milieu, will promote their development and yield greater populations of potential β-cells for further maturation upon implantation. In addition, pre-vascularization of the tissue may facilitate graft anastomosis and improve survival rates of the cells in vivo.
We designed an in vitro culture system utilizing polymeric scaffolds which provide cells with mechanical support and three-dimensional (3D) architecture. Clusters of hESC derived pancreatic cells were embedded in a vascular niche composed of endothelial cells (ECs) and/or fibroblasts. We show that one week of culturing the clusters with ECs in the 3D environment enhanced PPs’ marker expression (Pdx1, Nkx6.1, insulin) indicating an increase in PP population. Moreover, a marker indicating continued differentiation and commitment to endocrine fate (Ngn3) was significantly elevated in the presence of ECs. In addition, such constructs were able to protect mice against streptozotocin (STZ)-induced hyperglycemia.
Methods
Scaffold fabrication
3D scaffolds were prepared using a salt leaching technique,16 blending poly(lactide-co-glycolide) (PLGA; Boehringer-Ingelheim) and poly-L-lactic acid (PLLA; Polysciences) in 1
:
1 ratio, yielding a final pore size range of 300–600 μm and 93% porosity.17 These pore sizes were chosen to facilitate seeding and growth of both cell clusters and ECs. Sponges were cut into circular pieces of 6 mm diameter and sterilized in 70% ethanol before use.
Cell culture
Human umbilical vein endothelial cells expressing green fluorescent protein (HUVEC-GFP, passages 5–8, Angio-Proteomie, USA) were cultured in endothelial cell medium (EGM-2) supplemented with 5% fetal bovine serum (FBS, Hyclone, USA) and endothelial cell growth medium BulletKit (Lonza, USA).
Human neonatal dermal fibroblasts expressing green fluorescent protein (HNDF-GFP, passages 5–8, Angio-Proteomie, USA) were cultured in DMEM (Gibco, USA) supplemented with 5% FBS, 1% nonessential amino acids (Gibco, USA), 0.2% β-mercaptoethanol (Gibco, USA), and 1% Pen-Strep solution (Biological Industries, Israel).
Clusters of hESC (H1 line) derived pancreatic cells (Betalogics, NJ, USA) were generated using a previously published protocol18 and cultured in DMEM supplemented with 1% B27 (Gibco, USA) and 1% gentamicin (Sigma).
3D cluster culture
Four cellular cultures were seeded into scaffolds; all including 1 × 106 hESC derived pancreatic cells aggregated in 100–300 micron diameter clusters:
(i) Tri-culture: clusters, 0.5 × 106 HUVEC-GFP and 0.1 × 106 HNDF-GFP.
(ii) Co-culture with HUVEC-GFP: clusters with 0.5 × 106 HUVEC-GFP.
(iii) Co-culture with HNDF-GFP: clusters with 0.1 × 106 HNDF-GFP.
(iv) Clusters alone.
Cultures were prepared by suspending an appropriate cell combination in 10 μl of fibrin made of a mixture of 15 mg ml−1 fibrinogen (Sigma, Israel) and 50 U ml−1 Thrombin (Sigma, Israel) at 1
:
1 ratio. These cell mixtures were seeded into PLLA/PLGA scaffolds. Cell-embedded scaffolds were cultured in 50% endothelial medium and 50% DMEM-HG + 1% B27 medium for up to two weeks in a 5% CO2 humidified atmosphere at 37 °C.
Cultures of hESC-derived pancreatic cells smaller than 100 μm in diameter and single cells were seeded in the same manner. Small clusters were formed by intensive pipetting followed by filtration with a 100 μm filter and single cells were disassembled by incubation in TrypLE followed by pipetting and filtration with a 40 μm filter.
Immunofluorescence staining
Cultures were fixed in 4% PFA (EMS, PA, USA) for 10 min, washed with PBS and kept overnight in 30% sucrose at 4 °C. The next day samples were embedded in O.C.T., frozen and sectioned (30 μm thick). Immunofluorescence staining was performed as previously described. Primary antibodies used: polyclonal guinea-pig anti-Insulin (1
:
1400, Dako), rabbit anti C-peptide (1
:
100, Cell signaling), mouse anti glucagon (1
:
1400, Sigma) and polyclonal sheep anti-Ngn3 (1
:
400, R&D). After extensive washing, sections were incubated with secondary antibodies: donkey anti-guinea pig (1
:
500, Jackson ImmunoResearch), donkey anti-rabbit (1
:
400, Invitrogen), donkey anti-mouse (1
:
500, Invitrogen) and donkey anti-sheep (1
:
400; Invitrogen). Nuclei were counterstained using Dapi (1
:
1000, Sigma-Aldrich). Whole-mount 3D scaffolds and suspended clusters were fixated using 4% PFA, permeabilized with 0.3% Triton X-100 and labeled with polyclonal guinea-pig anti-Insulin (1
:
1000, Dako), polyclonal rabbit anti-Pdx1 (1
:
1000, Abcam) and monoclonal mouse anti-Nkx6.1 (1
:
400, University of Iowa). After extensive washing, scaffolds were incubated with donkey anti-guinea pig (1
:
500, Jackson ImmunoResearch), donkey anti-rabbit (1
:
500, Invitrogen) and donkey anti-mouse (1
:
400, Invitrogen). Nuclei were counterstained using Dapi (1
:
1000, Sigma-Aldrich).
Stained sections and whole mount samples were viewed using the Leica TCS LSI super-zoom macro confocal microscope.
Image analysis
Confocal images were analyzed using the Imaris image analysis software. Analysis was performed on original 3D images. Nuclear staining channels (Dapi, Pdx1, Nkx6.1, Ngn3, GFP) were converted to spot images composed of 8 μm spheres representing nuclei. Spots with centers closer than 10 μm from each other were considered to be overlapping. To distinguish the hESC-derived pancreatic cell population, GFP nuclei were subtracted from the total nuclei population, represented by the Dapi image. Insulin staining was converted to a surface since it is cytoplasmic. Nuclei closer than 5 μm to the surface were considered positive for insulin. Marker expression percentage was defined as the percent of pancreatic cells positive for a certain marker (overlapping spots or spots near the insulin surface) out of the entire pancreatic population.
Implantation of constructs and streptozotocin (STZ) treatment of mice
All animal experiments were approved by the Technion Institutional Care and Use Committee. Nude mice (Harlan) 8 weeks of age were anesthetized by intra-peritoneal injection of ketamine and xylazine (80 and 10 mg kg−1, respectively). A single implant construct (∼10 mm diameter) containing clusters only (4 × 106) or clusters + ECs (4 × 106 and 2 × 106 respectively) grown for 7 days in vitro was implanted into the subcutaneous space of the dorsal area.
Mice were grown for 3 months, after which they were treated with STZ in order to deplete their endogenous β-cells. Mice received 190 mg STZ (Sigma-Aldrich) per kg body weight, by single intra-peritoneal injection. For blood glucose measurements, tail vein samples were tested using a glucose oxidase-based monitor (MediSense Precision Plus, Abbott Laboratories, Doncaster, Victoria, Australia).
Statistical analysis
All statistics were performed using the GraphPad Prism software (GraphPad Software, La Jolla, CA). Results were analyzed using one-way ANOVA followed by a post hoc Tukey's Multiple Comparisons test. Results are expressed as mean ± standard error of the mean (SEM). We considered a probability value of ≤0.01 to be statistically significant (p < 0.01 = *, p < 0.001 = **, p < 0.0001 = ***).
Results
Engineering 3D constructs seeded with hESC derived pancreatic cell clusters
In order to more closely approximate the in vivo conditions, in vitro tissue engineering techniques were utilized to design 3D constructs based on the use of highly porous polymeric scaffolds.19 Clusters were embedded in PLLA/PLGA scaffolds together with human umbilical vein endothelial cells (HUVECs) and human neonatal dermal fibroblasts (HNDF) (Fig. 1A). Cells were seeded in a fibrin gel, a biological protein which helps facilitate cellular integration and growth on synthetic scaffolds. The combination of polymeric scaffolds and fibrin has been shown to sustain the tissue shape and mechanical properties, thanks to the presence of the scaffold, while fibrin supports biological activity but cannot withstand cell contraction forces on its own.20 Several fibrin densities and cell concentrations were tested to determine an optimal combination leading to self-assembly of mature intricate vessel networks in a matter of days (ESI, Fig. 1†). Confocal images of live cultures on days 2, 6 and 14 demonstrate the process of vessel network formation in the presence of clusters for the chosen fibrin gel and cell concentrations, from individual cells on day 2 to an interconnected mesh on days 7 and 14 (Fig. 1B). In addition, different configurations of hESC-derived pancreatic cells were examined to test whether the cells function better when subjected to cell–cell interactions, such as those found in dense clusters, or rather to contact with endothelial cells (ECs) which could be maximized by disassembling the clusters. Single pancreatic cells and clusters of various sizes were tested for this purpose. Pancreatic identity, indicated by pancreatic marker expression, was preserved in relatively large clusters (>100 micron diameter), whereas smaller cell aggregates and single cells ceased to express the markers almost completely (ESI, Fig. 2†). A confocal image of the larger clusters chosen, embedded in a 14-day-old vessel network composed of HUVEC-GFP and HNDF, exhibits the final design of our 3D, multicellular system (Fig. 1C).
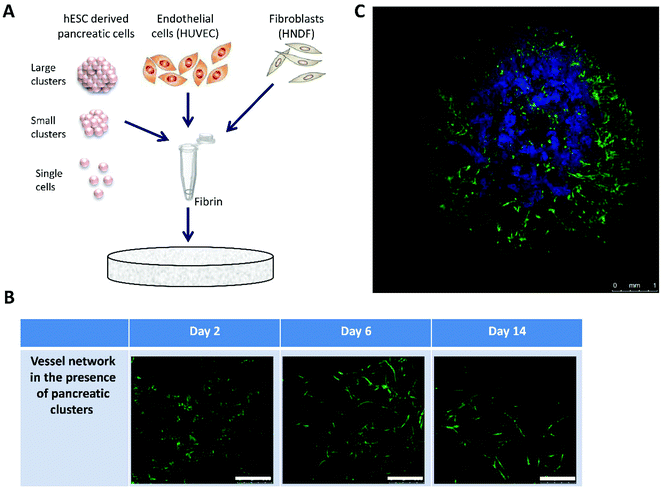 |
| Fig. 1 Design of a 3D multicellular construct for cultivation of pancreatic islets: (A) a general scheme depicting the seeding procedure of fibrin immersed multicellular cell preparations, including endothelial cells (ECs), fibroblasts and different configurations of human embryonic stem cell derived pancreatic cells, onto a polymeric (PLLA/PLGA) scaffold. (B) During the two weeks following seeding green fluorescent protein expressing ECs (HUVEC-GFP) and fibroblasts (HNDF) form a vessel network surrounding the pancreatic clusters (scale bars = 500 μm). (C) A confocal image of the final construct composed of clusters embedded in a 14-day-old vessel network established on the scaffold. | |
Morphological changes in clusters under different conditions
Clusters were seeded on scaffolds in the presence and absence of HUVEC-GFP and/or HNDF-GFP. Clusters in suspension served as a control for the two week experiment. Samples from each culture condition were fixed at three time points (days 2, 7 and 14), stained for pancreatic markers and imaged by confocal microscopy. Morphological changes in cluster size and shape were detected in whole-mount images (Fig. 2). In the presence of fibroblasts (Fig. 2A and B, left and center columns) an invasion of cells into the area of the cluster was seen on day 7 and increased by day 14. The invading cells were identified as HNDFs since this phenomenon did not appear in co-culture of ECs alone (Fig. 2A and B, right column). The fibroblasts rapidly proliferate and penetrate the clusters, breaking them up into smaller amorphous aggregates, entrapped inside the fibrotic tissue formed. Minimal proliferation of HUVEC-GFP was noted in the cluster/EC co-culture. Furthermore, a gradual disappearance of ECs was observed from day 2 to day 7. Clusters cultured with ECs alone maintained relatively constant shape and size, much like clusters alone on a scaffold (Fig. 2C, left column). Under both conditions the clusters spread throughout the scaffold and fused with neighboring clusters, while preserving the borders of each cluster. Cluster aggregates are also seen in suspension (Fig. 2C, right column); however, only minute alterations appear in the clusters’ spherical shape.
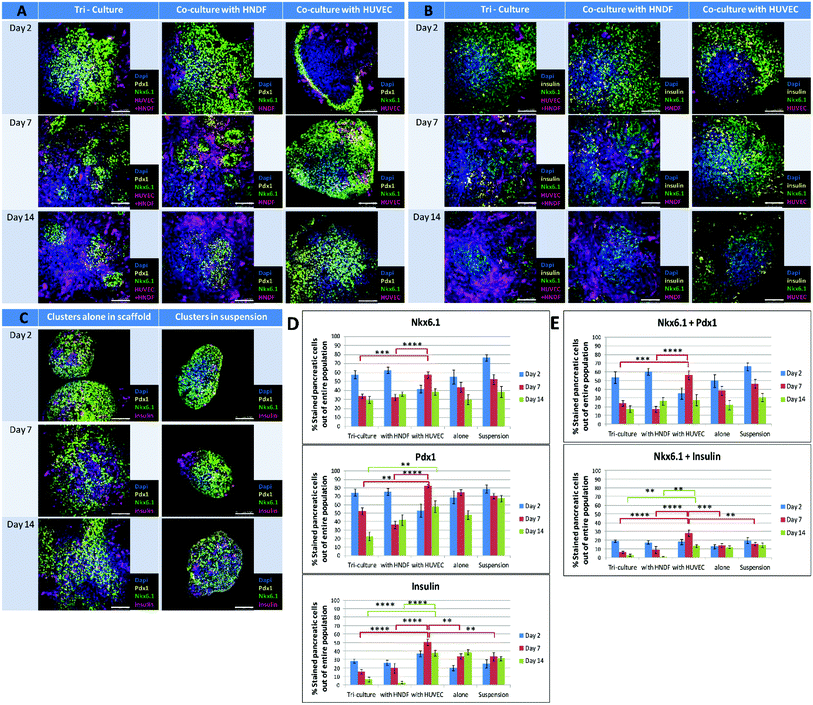 |
| Fig. 2 Pancreatic marker expressions in clusters cultured under different conditions: confocal images of immunofluorescence staining for pancreatic markers performed on whole constructs at 3 time points throughout the course of the experiment (days 2, 7 and 14) and respective quantification of marker expression in the images. Constructs contain pancreatic clusters cultured under five different conditions: HUVEC-GFP and HNDF-GFP tri-cultured or individually co-cultured with clusters appear in magenta (A and B). Tri-cultures and co-cultures were stained for either Pdx1 (yellow) and Nkx6.1 (green) (A) or for insulin (yellow) and Nkx6.1 (green) (B). (C) Controls, including clusters in suspension and alone on a scaffold, are stained for insulin (magenta), Pdx1 (yellow) and Nkx6.1 (green). All nuclei were stained with Dapi (blue). Scale bars for all images = 100 μm. The Imaris software was used to quantify the percentage of positive stained pancreatic cells out of the entire pancreatic cell population for the markers Nkx6.1, Pdx1 and insulin (D) and marker combinations of Nkx6.1 + Pdx1 and Nkx6.1 + insulin (E). Each bar represents the average percentage of marker positive cells, obtained from analyzing 8–20 confocal images of immunofluorescence staining for a specific condition in a certain day. Error bars indicate the standard error of the mean (SEM). Every set of three adjacent bars (blue–red–green) displays changes in the marker positive cell population of the condition marked below throughout the course of the experiment (days 2–7–14 respectively). Full statistical data are presented in ESI 3.† | |
Marker expression in clusters under different conditions
Initially, 90% of cells in the clusters express Pdx1, a key marker of early PPs.21 About 50–60% of cells are considered PPs, due mainly to co-expression of Pdx1 and Nkx6.1, a more specific PP marker.21 Expression of insulin in the Pdx1/Nkx6.1 positive cells appeared in further differentiated cells.9 The effect of different culture conditions was examined by measuring the expression of key pancreatic progenitors’ markers, such as Pdx1, Nkx6.1 and insulin, on days 2, 7 and 14 (Fig. 2). In controls (clusters in suspension or alone in a scaffold) Pdx1 and insulin expression was maintained, but a slight decrease was noted for Nkx6.1. Starting on day 7 distinct domains of insulin positive regions and Pdx1/Nkx6.1 co-expression appear (Fig. 2C). Clusters cultured with fibroblasts in tri- or co-cultures showed a decrease in all markers examined, especially insulin, which nearly disappeared by day 14 (Fig. 2B and D, bottom graph). In co-cultures of clusters with ECs (Fig. 2A, B, D and E), day 2 expression levels of all markers were particularly low, probably due to a unique occurrence observed at this time, where marker expression is found only on the outskirts adjacent to the edges of the clusters. However, this phenomenon is no longer seen on days 7 and 14, and markers reappear in all areas of the cluster. Moreover, on day 7 a distinctive increase occurs in all marker expression levels, which become the highest of all groups tested and in many cases even surpass expression levels recorded on day 2. The effect declines by day 14 and expression levels return to values similar to those of controls at the same day. The day 7 results indicate that the presence of ECs boosts the PP population compared to other cell-containing culture systems, and allows cell development towards β-cell differentiation.
Co-culturing clusters with ECs elevates Ngn3 expression on day 7
To evaluate whether beyond increasing PP population, a one week co-culture with ECs could also advance cell differentiation towards endocrine fate, detection of Ngn3 was performed. Ngn3 is the key marker for the transition of PPs to endocrine progenitors, the next stage of maturation towards β-cells.22 Immunofluorescence staining for insulin and Ngn3 was performed (Fig. 3A); images were quantified to present the percentage of Ngn3+ and Ngn3+/insulin+ cells (Fig. 3B and C). Images of all conditions except for EC co-culture show only a few stained cells, which are barely recognizable. Clusters cultured with ECs, on the other hand, contain many, easy to distinguish, stained cells. Furthermore, quantification shows a more than 3 fold increase in Ngn3+ cells in the cluster/EC co-culture compared to controls (3.3 folds, 232% higher), and a 4 fold increase in the case of Ngn3+/insulin+ cells (4.1 folds, 310% higher). Both trends are significant statistically (p < 0.001). These outcomes reinforce our findings of a positive effect on PP generation and further commitment to endocrine lineage, accomplished by cultivating hESC-derived pancreatic cell clusters in a 3D construct along with ECs.
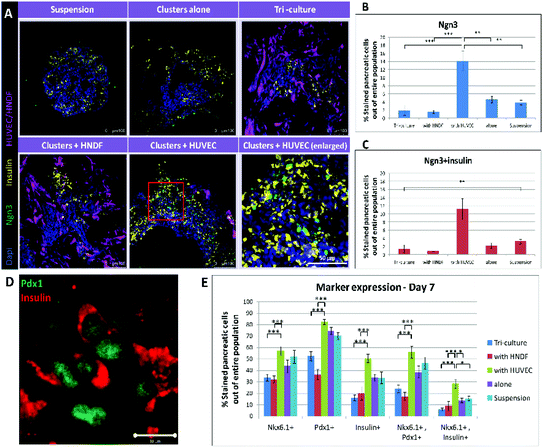 |
| Fig. 3 Co-culturing clusters with ECs leads to increased expression of Ngn3 and overall marker expression on day 7: (A) cryo-sections (30 μm thick) of constructs cultured under each condition (clusters in suspension, alone on a scaffold, tri-cultured with HUVEC + HNDF and co-cultured with HNDF or HUVEC) for 7 days were stained for Ngn3 (green) and insulin (yellow). Bottom right image shows an enlargement of the co-culture with the HUVEC image (red boxed area) demonstrating cells co-expressing Ngn3 and insulin. HUVEC-GFP and HNDF-GFP appear magenta in images. Dapi (blue) marks cell nuclei. (B–C) A quantification of the confocal in (A) was performed to obtain percentages of Ngn3 (B) and Ngn3 + insulin (C) positive cells out of the entire pancreatic cell population. Each bar represents an average percentage (n = 4) and error bars indicate the standard error of the mean (SEM). The value found for Ngn3 expression in the EC co-culture was significantly higher (>3-fold, p < 0.001) than under any other condition and for Ngn3 + insulin positive cells the effect was even greater (>4-fold, p < 0.001). (D) Higher magnification image illustrating co-expression of insulin (red) and Pdx1 (green) in cells. Bar = 10 μm. (E) A focus on all marker expressions on day 7. The percentages of pancreatic cells positive for: (from left to right) Nkx6.1, Pdx1, insulin, Nkx6.1 + Pdx1 and Nkx6.1 + insulin under every condition tested, as shown in Fig. 2. Levels of marker expressions in the co-culture with the EC group are maximal for all markers and marker combinations examined. These values are all significantly higher compared to fibroblast containing conditions (p < 0.0001), but only Nkx6.1 + insulin co-expression is significantly higher than controls (PPs alone and in suspension, p < 0.01). | |
Co-culturing clusters and ECs in 3D constructs for 7 days improves prospects of efficient β-cell replacement
The total effect seen after one week of culturing clusters with ECs in 3D scaffolds is greatly encouraging (Fig. 3E). Although the total number of cells positive for Pdx1 or Nkx6.1 did not rise significantly compared to controls (close to 14% and 20% increase of positive cells respectively), the number of cells co-expressing the two rose by 30% after a 7 day co-culture with ECs. Furthermore, the number of insulin positive cells was much higher with ECs (50% more than controls) and co-expression of Nkx6.1 and insulin showed an amazing increase of 91%. Compared to conditions including fibroblasts, all marker expression positive cells in the EC co-culture were significantly higher. These results are encouraging as the amount of cells co-expressing markers is an important factor, since it indicates how many cells have a high probability of developing into proper β-cells in vivo.
Implantation of constructs and streptozotocin (STZ) treatment of mice
To test the potential of the 3D constructs containing clusters and ECs grown for 7 days in vitro in regulating blood glucose levels independently of the endogenous β-cells, we conducted an in vivo study (Fig. 4). Mice were implanted with constructs containing clusters alone and clusters + ECs. Un-implanted mice served as a control. 3 months post-implantation, which allowed the pancreatic progenitors to mature, we treated the mice with STZ and then tested their blood glucose levels (Fig. 4A). While un-implanted mice became diabetic (as indicated by non-fasting glucose level higher than 300 mg dl−1) the blood glucose levels of both mice implanted with constructs containing clusters and clusters + ECs remained normal (∼130 mg dl−1). 5 weeks post-implantation, immunofluorescence staining of graft containing clusters + ECs (Fig. 4B) confirmed the presence of insulin secreting cells alongside glucagon secreting cells.
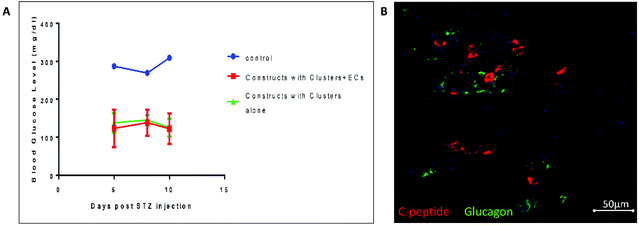 |
| Fig. 4
In vivo results – implantation of constructs grown for 7 days in vitro (A) 3 months post-implantation mice were treated with STZ. Non-fasting blood glucose levels are shown for mice implanted with constructs containing clusters alone or clusters + EC (n = 2) vs. un-implanted mice (control, n = 1). Each bar represents the average and error bars indicate the standard error of the mean (SEM). (B) Immunofluorescence staining of C-peptide (red) and glucagon (green) of implanted construct containing clusters + EC 5 weeks post-implantation. | |
Discussion
In the present study 3D multicellular constructs, composed of polymeric scaffolds and different combinations of ECs and fibroblasts, were designed (Fig. 1) in order to establish an optimal system for cultivating hESC-derived pancreatic cell clusters prior to transplantation. The main functions of these constructs are to support and perhaps also advance development of the clusters in vitro as well as to facilitate integration and function of the graft upon transplantation. The hypothesis underlying our research is that the 3D setting, mimicking natural in vivo conditions, together with blood vessels, which self-assemble in such constructs,19 will direct stem cell differentiation towards β-cells, in resemblance to the process occurring during actual embryogenesis.12–15 Construct design was based on previous work done in our lab, demonstrating formation of vascular niches composed of scaffolds, ECs and fibroblasts,20 and the positive influence such surroundings have on cultivation of mural pancreatic islets in vitro and in vivo.23 Tri-culturing ECs and fibroblasts with the clusters allowed the formation of vessel-like networks around the embedded clusters (Fig. 1C). However, substantial shape and size alterations were recorded for tri-cultured clusters (Fig. 2B and C). By observing co-cultures of clusters with ECs alone and fibroblasts alone (Fig. 2B and C) it was obvious that fibroblasts are responsible for this phenomenon. The fibroblasts penetrate the cluster, breaking it down to smaller aggregates and encapsulating them, while diminishing insulin production (Fig. 3A). Hence, despite their important role in vessel network formation, in our culture system fibroblasts, due to their extensive proliferation capacities, cause greater damage than gained from the vessels. Co-culturing mouse ESCs with ECs in the final stages of endocrine differentiation has already been shown to assist maturation into endocrine cells.24 In our study, addition of ECs to the cluster embedded structure resulted in two separate effects: limited marker expression confined to cluster edges on day 2 followed by a leap in expression and co-expression of all markers on day 7 (Fig. 3B and C). The effect recorded on day 2 resembles results presented by Talavera-Adame et al.25 who reported that co-culturing mouse embryonic bodies (EBs) with ECs caused increased expression of several pancreatic markers (among them Pdx1, Nkx6.1 and Ngn3) at the EB–EC interface, i.e. the periphery of EBs. The group observed marker expression strictly on the outskirts adjacent to the edges of the clusters of EBs following a 10 day co-culture with ECs. In contrast, our research showed a regain of marker expression on days 7 and 14 that was apparent all across the cluster, not only at the periphery. Nevertheless, similar to our results, Talavera-Adame et al. did not see increased expression when co-culturing the EBs with fibroblasts. The enhancement we saw in marker expression on day 7 faded by day 14, in parallel to an observed disappearance of HUVEC. ECs which are not dense enough tend to perish in the lack of supporting cells (for example fibroblasts).26 Thus, we suggest there is a tradeoff in our system; in the presence of fibroblasts clusters suffer from a fibroblast takeover, but in their absence ECs do not survive and their positive effect lasts only a short while. A possible resolution to this matter may be to enhance the amount of ECs seeded. Following studies will attempt to test whether higher concentrations of HUVEC intensify the positive effect seen on day 7 or if there is an optimal HUVEC concentration for culturing the clusters.
Beyond the elevation in PP marker expression seen in clusters co-cultured with ECs on day 7, indicating a large population of cells on the path of becoming endocrine cells, an additional trend was discovered by detection of Ngn3 expressing cells. Ngn3 is a key indicator of the commitment of cells to endocrine identity.22 A tremendous increase in Ngn3+ and Ngn3+/insulin+ cells was observed in the EC co-culture on day 7 (Fig. 3), marking the generation of a significant endocrine progenitor population unfound in any other condition at that time point. This finding demonstrates the contribution of the ECs to the development and differentiation of the clustered cells. It shows that the ECs in the culture not only boost the existing PP population, but they also induce cell differentiation into the next stage of maturation.
A definite trend emerges from observing marker expression levels of all examined culture conditions on day 7 (Fig. 3E); there is an obvious advantage in culturing pancreatic clusters in a 3D construct combining ECs. Even though individual markers (Pdx1, Nkx6.1, insulin) are not significantly elevated, all increase compared to controls (clusters alone on a scaffold or in suspension) and greatly compared to tri-culture or co-culture with fibroblasts. Nonetheless, the most meaningful factor is the significant increase in the amount of cells co-expressing markers, because this is the population from which β-cells will eventually evolve in vivo. β-Cell development is characterized by co-localization of pancreatic transcription factor expression areas and insulin production regions,9 thus separation of the two zones, such as seen in controls, may indicate cells evolving into other pancreatic cell types. However, in clusters co-cultured with ECs marker co-expressions are highly elevated, especially co-expression of insulin and Nkx6.1, indicating the existence of a large PP population. This outcome, together with the evidence of a significant endocrine progenitor population generated, may suggest that co-culturing pancreatic clusters together with ECs on our 3D construct will yield a greater amount of mature β-cells upon transplantation. To test this assumption an in vivo study was conducted (Fig. 4). 3 months post-implantation all implanted mice (with construct containing clusters + ECs and clusters alone) treated with STZ retained their blood glucose levels normal while un-implanted mouse became diabetic. This result is in accordance with previous work showing subcutaneous implantation of such cells inside a Theracyte device.27 Of note, 5 weeks post-implantation single hormonal cells expressing insulin or glucagon were observed (Fig. 4B), suggesting that the cells are developing towards a more mature form. The results show that PPs implanted in 3D constructs can survive, mature and function following in vitro maturation on EC containing scaffolds. However, in this set of in vivo studies we did not observe differences between culturing with or without EC. This can originate from the transient effect of ECs on in vitro maturation of PPs, or suggest that higher levels of EC are required to enhance maturation and survival of PPs in vivo.
It is important to mention that since the effect of EC on PP differentiation seems transient, longer culture on scaffolds may be non-beneficial, and rather shorter maturation on scaffolds could provide better results in enhancing in vivo maturation. Since cell recovery from the scaffolds is currently inefficient, maturation is performed on the same scaffolds that are implanted, thus co-cultures must be designed in advance to allow the right in vitro and in vivo support.
Acknowledgements
This research was supported by the ISF – JDRF Joint Program in Type I Diabetes Research (grant No. 1663/11). The research leading to these results has received funding from the European Research Council under the European Union's Seventh Framework Programme (FP/2007–2013)/ERC Grant Agreement no. 281501. This study was also partially supported by the Russell Berrie Nanotechnology Institute, Technion, Israel Institute of Technology and in part at the Technion by a fellowship from the Lady Davis Foundation. The authors would like to thank Janette Zavin for preparing samples to immunofluorescence analysis, to Galia Ben-David for her technical support, to the staff of the Technion Animal House Care Facility and to the staff of the Technion LS & E Infrastructure Unit.
References
- A. M. Shapiro,
et al., Islet transplantation in seven patients with type 1 diabetes mellitus using a glucocorticoid-free immunosuppressive regimen, N. Engl. J. Med., 2000, 343, 230–238 CrossRef CAS PubMed.
- R. G. Bretzel,
et al., Islet cell transplantation today, Langenbecks Arch. Surg., 2007, 392, 239–253 CrossRef PubMed.
- F. W. Pagliuca and D. A. Melton, How to make a functional β-cell, Development, 2013, 140, 2472–2483 CrossRef CAS PubMed.
- G. Kolios and Y. Moodley, Introduction to stem cells and regenerative medicine, Respiration, 2013, 85, 3–10 CrossRef PubMed.
- K. A. D'Amour,
et al., Production of pancreatic hormone-expressing endocrine cells from human embryonic stem cells, Nat. Biotechnol., 2006, 24, 1392–1401 CrossRef PubMed.
- D. Zhang,
et al., Highly efficient differentiation of human ES cells and iPS cells into mature pancreatic insulin-producing cells, Cell Res., 2009, 19, 429–438 CrossRef CAS PubMed.
- E. Kroon,
et al., Pancreatic endoderm derived from human embryonic stem cells generates glucose-responsive insulin-secreting cells in vivo, Nat. Biotechnol., 2008, 26, 443–452 CrossRef CAS PubMed.
- J. Jiang,
et al., Generation of insulin-producing islet-like clusters from human embryonic stem cells, Stem Cells, 2007, 25, 1940–1953 CrossRef CAS PubMed.
- A. Rezania,
et al., Maturation of human embryonic stem cell-derived pancreatic progenitors into functional islets capable of treating pre-existing diabetes in mice, Diabetes, 2012, 61, 2016–2029 CrossRef CAS PubMed.
- D. Nyqvist,
et al., Donor islet endothelial cells in pancreatic islet revascularization, Diabetes, 2011, 60, 2571–2577 CrossRef CAS PubMed.
- M. Brissova and A. C. Powers, Revascularization of transplanted islets: can it be improved?, Diabetes, 2008, 57, 2269–2271 CrossRef CAS PubMed.
- E. Lammert, O. Cleaver and D. Melton, Role of endothelial cells in early pancreas and liver development, Mech. Dev., 2003, 120, 59–64 CrossRef CAS.
- G. Nikolova,
et al., The vascular basement membrane: a niche for insulin gene expression and Beta cell proliferation, Dev. Cell, 2006, 10, 397–405 CrossRef CAS PubMed.
- O. Cleaver and Y. Dor, Vascular instruction of pancreas development, Development, 2012, 139, 2833–2843, DOI:10.1242/dev.065953.
- E. Lammert, O. Cleaver and D. Melton, Induction of pancreatic differentiation by signals from blood vessels, Science, 2001, 294, 564–567 CrossRef CAS PubMed.
- S. Levenberg,
et al., Engineering vascularized skeletal muscle tissue, Nat. Biotechnol., 2005, 23, 879–884 CrossRef CAS PubMed.
- S. Levenberg,
et al., Differentiation of human embryonic stem cells on three-dimensional polymer scaffolds, Proc. Natl. Acad. Sci. U. S. A., 2003, 100, 12741–12746 CrossRef CAS PubMed.
- J. E. Bruin,
et al., Maturation and function of human embryonic stem cell-derived pancreatic progenitors in macroencapsulation devices following transplant into mice, Diabetologia, 2013, 56, 1987–1998 CrossRef PubMed.
- S. Levenberg and R. Langer, Advances in tissue engineering, Curr. Top. Dev. Biol., 2004, 61, 113–134 CrossRef CAS.
- A. Lesman,
et al., Engineering vessel-like networks within multicellular fibrin-based constructs, Biomaterials, 2011, 32, 7856–7869 CrossRef CAS PubMed.
- G. K. Gittes, Developmental biology of the pancreas: a comprehensive review, Dev. Biol., 2009, 326, 4–35 CrossRef CAS PubMed.
- G. Gu, J. Dubauskaite and D. A. Melton, Direct evidence for the pancreatic lineage: NGN3+ cells are islet progenitors and are distinct from duct progenitors, Development, 2002, 129, 2447–2457 CAS.
- K. Kaufman-Francis, J. Koffler, N. Weinberg, Y. Dor and S. Levenberg, Engineered vascular beds provide key signals to pancreatic hormone-producing cells, PLoS One, 2012, 7, e40741 CAS.
- I. Banerjee, N. Sharma and M. Yarmush, Impact of co-culture on pancreatic differentiation of embryonic stem cells, J. Tissue Eng. Regener. Med., 2011, 5, 313–323 CrossRef CAS PubMed.
- D. Talavera-Adame,
et al., Endothelial cells in co-culture enhance embryonic stem cell differentiation to pancreatic progenitors and insulin-producing cells through BMP signaling, Stem Cell Rev., 2011, 7, 532–543 CrossRef CAS PubMed.
- D. Gospodarowicz, J. Moran, D. Braun and C. Birdwell, Clonal growth of bovine vascular endothelial cells: fibroblast growth factor as a survival agent, Proc. Natl. Acad. Sci. U. S. A., 1976, 73, 4120–4124 CrossRef CAS.
- E. J. Bruin, Maturation and function of human embryonic stem cell-derived pancreatic progenitors in macroencapsulation devices following transplant into mice, Diabetologia, 2013, 56, 1987–1998 CrossRef PubMed.
Footnote |
† Electronic supplementary information (ESI) available. See DOI: 10.1039/c4bm00304g |
|
This journal is © The Royal Society of Chemistry 2014 |
Click here to see how this site uses Cookies. View our privacy policy here.