DOI:
10.1039/C4LC00596A
(Paper)
Lab Chip, 2014,
14, 3505-3509
Multiplexed immunoassay based on micromotors and microscale tags†
Received
21st May 2014
, Accepted 30th June 2014
First published on 30th June 2014
Abstract
This work reports on the coupling of antibody-functionalized micromotors and microwire-tagged proteins for rapid and multiplexed immunoassays. While micromotor-induced mixing accelerates the immunoreaction, tagging the proteins with microscopic particles of different sizes and shapes allows for their multiplexed discrimination, alerting of the presence of a biological threat.
Introduction
Creation of deadly pathogenic microorganisms (and their metabolites) and biological warfare agents as new means of terrorism that threaten the health and security of the population has led to the accelerated development of biodetection technology.1,2 Critical to successful biothreat surveillance is the ability to screen for and detect multiple agents rapidly in a single step and with minimal sample processing.3 Giving an insight into a simple and rapid biodetection system of dangerous protein toxins, we report a new concept of a multiplexed immunoassay based on functionalized micromotors and microwire tags for the rapid and on the fly discrimination of proteins coexisting with other protein counterparts. The new motor-based multiplexed concept is demonstrated with ovalbumin as a simulant of protein toxins, solving the safety issue of manipulating dangerous threats or infectious organisms. The assay comprises antibody-functionalized bubble-propelled micromotors (as mobile biosensing platforms) that, along with protein-tagged microwires (serving as preconcentrators), are employed for the rapid visualization of the antigen–antibody binding event.
Here, we use bubble-powered tubular micromotors4–6 that exhibit autonomous self-propulsion in the presence of hydrogen peroxide fuel. Such micromotors have attracted remarkable interest due to their attractive cargo-towing and solution-mixing capabilities in diverse biomedical7–12 and environmental13,14 applications. The efficient motor-induced fluid mixing of such tubular microengines has shown to be extremely effective in accelerating both target–receptor15 and detoxification reactions.13,14 For efficient multiplexed detection, along with micromotors, the new immuno protocol also uses electroplated microscale materials of different shapes or sizes (wires or spheres).16,17 Whereas a variety of metals can be electroplated, we employed gold that facilitates the target analyte tagging process. Similarly, a microsphere coated with proteins has been used to represent the epitopes of a real microorganism. Different sizes of the tags offer a rapid and direct visualization of the recognition event and effective discrimination of other proteins.18 A detailed model of reaction kinetics has revealed that tagging the targets with microbeads in solution provides a significant kinetic advantage at low target concentrations where mass transport is limited. For instance, target collection provided a 1000-fold improvement in the sensitivity towards enterotoxin at fg ml−1 levels.19 Metallic striped nanowires have also been explored as multiplexed immunoassay platforms for pathogen detection,20 but not in connection with mobile antibody functionalized micromotors. Herein, tagging the analyte with a microparticle of different sizes and shapes offers the unique opportunity not only for the direct visualization of the antibody–protein recognition event but also for the efficient preconcentration of the protein onto a microstructure which will favor the multiplexed discrimination of the target, in agreement with previous reports.19 Whereas the micromotor/microwire-based multiplexed immunoassay is demonstrated here for a simulant of dangerous protein toxins, the concept can be extended to the development of simple and effective systems that rapidly differentiate other types of coexisting biomolecules (e.g. DNA) and whole systems conducted to alert us about the presence of a biological threat.
Experimental section
Synthesis of multilayer micromotors
The multilayer microtubes were prepared using a common template-directed electrodeposition protocol.21 A cyclopore polycarbonate membrane, containing 2 mm maximum diameter conical-shaped micropores (catalog no. 7060-2511; Whatman, Maidstone, UK), was employed as a template. A 75 nm gold film was first sputtered on one side of the porous membrane to serve as a working electrode using a Denton Discovery 18. Sputtering was performed at room temperature under a vacuum of 5 × 10−6 Torr and a DC power of 200 W and Ar was flowed at 3.1 mT. The rotation speed was 65 rpm along with a sputtering time of 90 s. A Pt wire and Ag/AgCl with 3 M KCl were used as counter and reference electrodes, respectively. The membrane was then assembled in a plating cell with aluminum foil serving as the contact for the working electrode. PEDOT/PEDOT–COOH microtubes were electropolymerized for a total charge of 0.15 C at +0.85 V from a plating solution containing 12 and 3 mM EDOT and EDOT–COOH monomer, 100 mM SDS and 7.5 mM KNO3; all of them were prepared from Sigma-Aldrich reagents. Then, the metallic layers were deposited from Pt and Ni solutions. A platinum commercial solution was employed (Platinum RTP; Technic Inc, Anaheim, CA) and nickel solution was prepared by 11 g l−1 NiCl2·6H2O, 300 g l−1 Ni(H2NSO3)2·4H2O, 30 g l−1 H3BO3 and 0.0488 g l−1 SDS. The first Pt layer was deposited galvanostatically at −2 mA for 500 s to provide a smooth and high conductive surface and also improve the deposition of the next metallic layers. After washing three times with water, the intermediate Ni layer was deposited amperometrically at −1.0 V and 1.8 C to achieve the magnetic properties allowing the micromotor guidance by properly orienting the magnetic field created with a simple neodymium magnet. Finally, after another three washing steps, the catalytic inner Pt layer was deposited galvanostatically at −2 mA for 350 s. To release the microengines from the template, the sputtered gold layer was completely removed using a mechanical hand polishing with 3–4 μm alumina slurry. The membrane was then dissolved in methylene chloride for 10 min to completely release the microtubes. Finally, microengines were washed repeatedly with methylene chloride, followed by washing twice with ethanol and ultrapure water (18.2 MU cm) and collected by centrifugation at 7000 rpm for 3 min after each wash.
Micromotor functionalization
1-Ethyl-3-(3-dimethylaminopropyl) carbodiimide (EDC)/N-hydroxysuccinimide (NHS) chemistry was used to activate the carboxyl-terminated groups from the polymer for conjugation with anti-immunoglobulin G (I5260, Sigma), anti-myoglobin (M8648, sigma), anti-thrombin (PA1-74128, Thermo Scientific) and anti-ovalbumin (T261002-01, Tetracore) proteins. For this purpose, micromotors were treated with 100 μl of 0.025 M MES buffer solution (pH 6.5), containing 20 mM EDC and 40 mM NHS for 30 min, washed with MES buffer for 3 min and incubated with each antibody (200 μg ml) in PBS 1× (pH 7.2) for 2 h. The excess of antibody was washed in 1× PBS buffer (pH 7.2) containing 0.05% Tween-20. The remaining amine-reactive esters from the activated carboxylic groups were blocked with 1 M ethanolamine solution, pH 8.5, for 20 min and 1% BSA for 1 hour with a washing step in between with 1× PBS and 0.05% Tween-20 (pH 7.2). In all the washing steps the micromotors were centrifuged at 7000 rpm for 3 min. All the experiments were carried out under gentle shaking at room temperature.
Microstructures functionalization
Different gold structures as wires (2 and 4 μm) and squares (2 μm × 600 μm) were used. The external gold surface of gold wires and squares was modified by overnight shaking in 1 ml of alkanethiol solution (0.25 mM 1-mercaptoundecanoic acid (MUA)/0.75 mM 6-mercaptohexanol (MCH) in ethanol). Afterward, the gold structures were washed with 100 μl of water. All carboxyl-terminated groups from the different microstructures were activated by EDC and NHS chemistry for conjugation with different proteins. 200 μg ml−1 of Human IgG (I4506, Sigma), Myoglobin (M1882, Sigma), Thrombin (T1063, Sigma) or Ovalbumin (A7641, Sigma) dissolved in 1× PBS (pH 7.2) were used following the protocol previously described for micromotor functionalization.
Equipment
Template electrochemical deposition of microtubes was carried out with a CHI 661D potentiostat (CH Instruments, Austin, TX). An inverted optical microscope (Nikon Eclipse Instrument Inc. Ti-S/L100), coupled with 20× and 40× objectives, along with a Hamamatsu digital camera C11440 and NIS-Elements AR 3.2 software, was used to capture movies at a frame rate of 10 frames per second.
Experimental procedure
An equal volume of micromotors, microwires, fuel and surfactant solutions is placed on a glass slide to get a final concentration of 5% sodium cholate (NaCh) and 3% H2O2 in 1× PBS buffer (pH 7.2) solution. Tracking of the micromotors was followed using the inverted optical microscope. Small quantities of either PBS or fuel were further added when necessary to ensure both the autonomous navigation of the micromotors over prolonged time periods and the appropriate speed.
Results and discussion
The micromotor-based multiplexed immunoassay consists of micromotors functionalized with different antibodies for the selective recognition and capture of different microscopic gold wire-tagged target proteins. Tagging the proteins with wires of different sizes and shapes allows not only for the direct visualization of the antigen–antibody recognition event, due to the microscopic size of the wires, but also for the selective and simultaneous recognition of the target proteins based on different sizes or shapes of the wires. Therefore, a significant kinetic advantage is expected from both the movement of micromotors22 and the preconcentration of the target protein onto the surface of the microwire tags.19Fig. 1A shows a sketch of the micromotors functionalized with 2 different antibodies reacting with 2 different target proteins tagged with spherical and cylindrical gold microstructures for their visual discrimination. It is important to note that the proteins can be either anchored directly on the microstructure surface (A, bottom part) or alternatively be anchored through a secondary antibody (A, upper part), analogous to a conventional sandwich immunoassay. Although the sandwich format is commonly used for practical applications,11,12 for simplicity, the former format was used in the present work to proof the multiplexing concept. Fig. 1B shows the EDC/NHS chemistry employed to anchor the proteins onto the surface of the microstructure tags functionalized with self-assembled monolayers (SAMs) of MUA and MCH. Amino moieties of the antibodies are covalently attached to the carboxylic groups of the outermost layer of the micromotor surface also by the EDC/NHS reaction. The immuno-micromotors were prepared by template-based electrodeposition of multilayers PEDOT:COOH–PEDOT/Ni/Pt microtubes as reported.11 Whereas the outermost polymeric layer is synthesized by co-electropolymerization of PEDOT:PEDOT–COOH from an electroplating solution containing a mixture of the monomers, the exposed carboxylic moieties are used for the covalent attachment of the antibodies. Electrodeposition of Ni and Pt metallic layers (from Pt and Ni electroplating solution, respectively) provides magnetic and catalytic properties for the magnetic guidance and propulsion of the microengines, respectively. Electrochemical deposition conditions were previously optimized11 (see details in Experimental section). The resulting 8–12 μm-long polymer/Ni/Pt microtubes propel efficiently via the ejection of oxygen bubbles generated by the catalytic decomposition of hydrogen peroxide fuel at their inner Pt layer5 at an ultra-fast speed of ~400 μm s−1. The micrometer size of the motors and nanowire tags offers a convenient real-time optical visualization of the protein binding event.
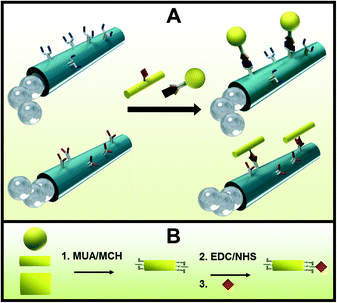 |
| Fig. 1 Scheme showing the micromotor/microwire-based multiplexed immunoassay for selective detection of proteins. PEDOT–COOH–PEDOT/Ni/Pt multilayer micromotors are functionalized with different antibodies (A), which recognize the corresponding antigens conjugated to microscale gold tags of different sizes or shapes (B). Antibodies are attached to the micromotors by linking their amino moieties to the carboxyl group at the outermost layer of the micromotor surface by means of the standard EDC/NHS chemistry. Antigens are tagged by using the same chemistry (EDC/NHS) after functionalization of the microwire tags with mixed SAMs of MUA/MCH. | |
To demonstrate the capability of directly detecting potential biological warfare agents, the micromotors are first functionalized with an anti-ovalbumin antibody and were allowed to navigate in a buffered solution containing tagged ovalbumin target proteins. Herein, we use the innocuous ovalbumin protein to simulate dangerous protein toxins such as ricin or botulinum, while handling such a biomaterial in the laboratory with minimal safety risk.18,19 Additionally, the use of microparticles coated with nanometric proteins, which represent the epitopes of real microorganisms, emulates such microscopic organisms completely avoiding their potential danger and controlling the epitope density, thus greatly facilitating the study of biological threats in terms of preparation, manipulation and storage. Fig. 2A(a–c) and ESI† Video 1 show an anti-ovalbumin antibody-functionalized micromotor approaching, contacting and carrying a 4 μm gold wire-tagged ovalbumin antigen. The results demonstrate that micromotors functionalized with selective receptors can rapidly interact with target proteins leading to ‘on the fly’ visualization of the recognition event for the simple and rapid identification of a potential biological threat. The approach has the added advantage of avoiding the common washing steps of standard immunoassays.
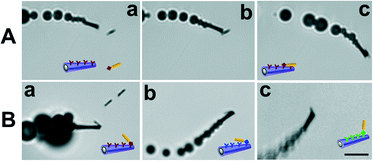 |
| Fig. 2 Detection of multiple proteins based on the micromotor/microwire-tag assay. A) Antibody-modified micromotor approaching (a), contacting (b) and carrying (c) a 4 μm ovalbumin-modified wire. B) A specific antibody-functionalized micromotor carrying the respective IgG (a), myoglobin (b) and thrombin (c) target proteins tagged with 4 μm wires. Motion conditions, a 1× PBS solution (pH 7.4) containing 3% H2O2, 5% NaCh. Scale bar, 10 μm. A sketch of the tagged proteins is included. | |
Towards proofing the multiplexing concept and expanding the scope of application, the assay was applied not only to other model proteins such as immunoglobulins (IgG), but also to some others of medical interest like myoglobin and thrombin. Fig. 2B(a–c) and ESI† Video 2 show micromotors coated with specific antibodies recognizing and picking up IgG, myoglobin and thrombin, which are previously tagged with 4 μm gold wires. Although in all cases the capture and transport of the tagged proteins by the functionalized micromotors were possible, interestingly, the efficiency of the recognition event was not always the same. For example, whereas the anti-IgG antibody-coated micromotors instantaneously captured the microwire-tagged IgG proteins in 90% of the contacts, anti-ovalbumin antibody-functionalized micromotors captured wire-tagged ovalbumin proteins in only 25% of the cases. In a similar manner, the efficiency of the antigen–antibody interactions for both myoglobin and thrombin was estimated to be around 15%. Although this observation is a priori in agreement with the different reaction kinetics expected for different antigen–antibody complexes23–25 and it is worth studying in detail, it is beyond the scope of this work. However, we hypothesize that the molecular composition of the proteins, e.g. number of amino moieties and specially their spatial location and availability when coupling to carboxylic groups (at micromotors and microwires surface), is also contributing to the different interaction efficiencies observed. The H2O2 fuel concentration might be also differently affecting the immunoreactions.15
Specificity is another important feature when testing the performance of antibodies in immunoreactions and it was tested using IgG as the model protein. ESI† Video 3 shows an anti-IgG antibody-functionalized micromotor picking up and transporting even 3 or 4 μm wire-tagged IgG proteins, while ‘bypassing’ an unmodified wire (without proteins; right) that is used as a negative control. Similarly, another negative control involved a micromotor without antibodies that bypasses wire-tagged IgG proteins. These results demonstrate that antibody-functionalized micromotors are able to only interact (pick up and transport) with the properly microobject-tagged proteins.
To test the potential of micromotor-based immunoassay for multiplexed detection we functionalized the micromotors with model anti-IgG antibodies and tagged the IgG target proteins with microparticles of different sizes and shapes. While Fig. 3A(a–c) illustrates a functionalized micromotor approaching, contacting and transporting a 4 μm gold wire, in Fig. 3B(a–c) the proteins are tagged with a 2 μm gold wire and in Fig. 3C(a–c) with a 2 μm gold sphere (see also the ESI,† Video 4). ESI† Fig. S1 shows an anti-IgG-modified micromotor approaching (left), contacting (center), and carrying (right) a 4 μm wire-tagged IgG protein (a) and bypassing a 2 μm unmodified wire (b) vs. a 2 μm unmodified wire (c) as the negative control, respectively. These results demonstrate that tagging the proteins with microparticles of different sizes and shapes is a unique approach for the direct and visual recognition of the target protein while avoiding the enzymatic labeling, commonly used in standard immunoassays as indication of the recognition event. Finally we demonstrated the feasibility of the micromotor-based multiplexed immunoassay towards selective detection of simulants of dangerous protein toxins. For this purpose, the micromotors are functionalized with antibodies selective to ovalbumin proteins, the biowarfare agent simulants, and navigated in a buffered solution containing a mixture of both the ovalbumin target and the IgG proteins, both of them tagged with microparticles of different sizes and shapes. Fig. 4A and ESI† Video 5 illustrate the multiplexed discrimination of the target toxin. An anti-ovalbumin antibody-coated micromotor captures and transports 4 μm microwire-tagged ovalbumin proteins after it approaches, contacts and bypasses 2 μm square-tagged IgG proteins. In a similar manner, an anti-IgG antibody-coated micromotor captures and transports 2 μm square-tagged IgG proteins after contacting and bypassing 4 μm wire-tagged ovalbumin proteins (Fig. 4B and ESI† Video 6). These results demonstrate that the multiplexed detection of proteins based on direct visualization of moving micromotors and microscale tags allows for the discrimination of multiple coexisting proteins. The concept can be easily implemented in the discrimination of real targets by means of a simple sandwich assay where the target is captured at the micromotor (or microobject tag) surface and the recognition event indirectly visualized by subsequent capturing of a secondary-tagged antibody. Results also demonstrate that proteins can be tagged with diverse microparticles of multiple sizes and shapes to preconcentrate the analyte and that the size of the microparticle is not crucial for the tagging as soon as they are distinguishable under the microscope. The new versatile approach opens up an opportunity for discriminating against target proteins and holds promise as a screening method that can rapidly alert us of the presence of a biowarfare threat in a real-time simple fashion.
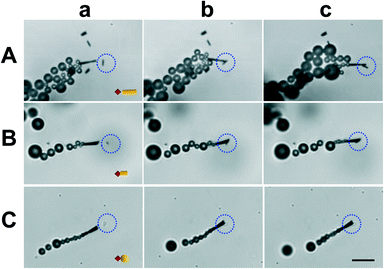 |
| Fig. 3 Motor-based multiplexed protein immunoassay, IgG tagged with particle tags of different shapes and sizes. Anti-IgG-modified micromotor approaching (a), contacting (b) and carrying (c) IgG proteins tagged with a 4 μm wire (A), a 2 μm wire (B) and a 2 μm sphere (C), respectively. Scale bar, 10 μm. Conditions, as in Fig. 2. The tagged analyte is highlighted with a blue dotted circle. A sketch of the tagged proteins is included in (a). | |
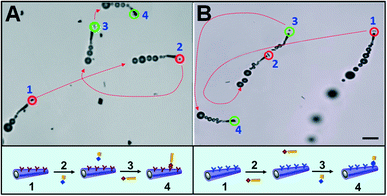 |
| Fig. 4 Multiplexed detection of proteins. An anti-ovalbumin-functionalized micromotor capturing 4 μm wire-tagged ovalbumin proteins after bypassing 2 μm square-tagged IgG proteins (A) and an anti-IgG functionalized micromotor capturing 2 μm square-tagged IgG proteins after ‘bypassing’ 4 μm wire-tagged ovalbumin proteins (B). Scale bar, 10 μm. Conditions, as in Fig. 2. Dotted red lines indicate the trajectory of the moving functionalized micromotors. Targets and non-targets are highlighted with green and red circles, respectively. (a–d) Indicate a functionalized micromotor (a) approaching to and bypassing the tagged non-target protein (b), and approaching to (c) and carrying the tagged target protein (d). A sketch of the operation performed by the micromotor is shown in the bottom part from (a)–(d). | |
Conclusions
We have demonstrated the potential of a micromotor/microwire-based multiplexed immunoassay for the discrimination of toxic proteins in real time. The functional micromotors selectively recognized the tagged proteins based on the shape and (or) size of microscopic tags. A simulant of a dangerous protein toxin was captured and accumulated on a microobject surface and detected by simple visual observation. In addition, the protein-coated microtags mimic pathogens, thus demonstrating that safety, easy handling and storage benefits of this approach can also be achieved in screening for microorganisms. Such outstanding capabilities are advantageous in a variety of bioassays with respect to the reported self-propelled micromagnet counterparts.26 We can envision a microfluidic device incorporating the new micromotor/microwire-based multiplexed immunoassay, where capture, transport, tagging, cleanup, enrichment, detection and release multiple operations can be integrated within spatially separated zones.27,28 It allows for a portable biosensing system that alerts of the presence of biological warfare agents among other interesting applications.
Acknowledgements
This project received support from the Defense Threat Reduction Agency–Joint Science and Technology Office for Chemical and Biological Defense (HDTRA1-13-1-0002). D.V. and G.C. acknowledge financial support from the Spanish Science and Innovation Ministry and the China Scholarship Council, respectively.
Notes and references
- H. C. Lane, J. L. Montagne and A. S. Fauci, Nat. Med., 2001, 7, 1271 CrossRef CAS PubMed.
- B. Durodie, Curr. Opin. Biotechnol., 2004, 15, 264 CrossRef CAS PubMed.
- N. M. Cirino, K. A. Musser and C. Egan, Expert Rev. Mol. Diagn., 2004, 4, 841 CrossRef CAS PubMed.
- Y. F. Mei, G. S. Huang, A. A. Solovev, E. B. Urena, I. Monch, F. Ding, T. Reindl, R. K. Y. Fu, P. K. Chu and O. G. Schmidt, Adv. Mater., 2008, 20, 4085 CrossRef CAS.
- W. Gao, S. Sattayasamitsathit, J. Orozco and J. Wang, J. Am. Chem. Soc., 2011, 133, 11862 CrossRef CAS PubMed.
- G. Zhao and M. Pumera, RSC Adv., 2013, 3, 3963 RSC.
- L. K. E. A. Abdelmohsen, F. Peng, Y. Tu and D. A. Wilson, J. Mater. Chem. B, 2014, 2, 2395 RSC.
- J. Wang and W. Gao, ACS Nano, 2012, 6, 5745 CrossRef CAS PubMed.
- S. Campuzano, J. Orozco, D. Kagan, M. Guix, W. Gao, S. Sattayasamitsathit, J. C. Claussen, A. Merkoci and J. Wang, Nano Lett., 2012, 12, 396 CrossRef CAS PubMed.
- A. A. Solovev, W. Xi, D. H. Gracias, S. M. Harazim, C. Deneke, S. Sanchezand and O. G. Schmidt, ACS Nano, 2012, 6, 1751 CrossRef CAS PubMed.
- M. García, J. Orozco, M. Guix, W. Gao, S. Sattayasamitsathit, A. Escarpa, A. Merkoçi and J. Wang, Nanoscale, 2013, 5, 1325 RSC.
- X. Yu, Y. Li, J. Wu and H. Ju, Anal. Chem., 2014, 86, 4501 CrossRef CAS PubMed.
- J. Orozco, G. Cheng, D. Vilela, S. Sattayasamitsathit, R. Vazquez-Duhalt, G. Valdes-Ramirez, O. S. Pak, A. Escarpa, C. Kan and J. Wang, Angew. Chem., Int. Ed., 2013, 52, 13276 CrossRef CAS PubMed.
- L. Soler, V. Magdanz, V. M. Fomin, S. Sanchezand and O. G. Schmidt, ACS Nano, 2013, 7, 9611 CrossRef CAS PubMed.
- E. Morales-Narváez, M. Guix, M. Medina-Sánchez, C. C. Mayorga-Martinez and A. Merkoçi, Small, 2014, 9, 2542 CrossRef PubMed.
- S. R. Nicewarner-Pena, R. G. Freeman, B. D. Reiss, L. He, D. J. Pena, I. D. Walton, R. Cromer, C. D. Keating and M. J. Natan, Science, 2001, 294, 137 CrossRef CAS PubMed.
- C. D. Keating and M. J. Natan, Adv. Mater., 2003, 15, 451 CrossRef CAS.
- C. SupapornKradtap, A. Wijayawardhana, K. T. Schlueter, H. Brian Halsall and W. R. Heineman, Anal. Chim. Acta, 2001, 444, 13 CrossRef.
- S. P. Mulvaney, K. M. Myers, P. E. Sheehan and L. J. Whitman, Biosens. Bioelectron., 2009, 24, 1109 CrossRef CAS PubMed.
- J. B. Tok, F. Y. Chuang, M. C. Kao, K. A. Rose, S. S. Pannu, M. Y. Sha, G. Chakarova, S. G. Penn and G. M. Dougherty, Angew. Chem., Int. Ed., 2006, 45, 6900 CrossRef CAS PubMed.
- W. Gao, S. Sattayasamitsathit, A. Uygun, A. Pei, A. Ponedal and J. Wang, Nanoscale, 2012, 4, 2447 RSC.
- J. Orozco, S. Campuzano, D. Kagan, M. Zhou, W. Gao and J. Wang, Anal. Chem., 2011, 83, 7962 CrossRef CAS PubMed.
- A. Sardana and D. Sii, Biosens. Bioelectron., 1992, 7, 559 CrossRef.
- M. Stenberg and H. Nygren, J. Immunol. Methods, 1988, 113, 3 CrossRef CAS.
- C. J. Van Oss, J. Chromatogr., 1986, 876, 111 CrossRef.
- G. Zhao, H. Wang, S. Sanchez, O. G. Schmidt and M. Pumera, Chem. Commun., 2013, 49, 5147 RSC.
- M. Pumera, Chem. Commun., 2011, 47, 5671 RSC.
- J. Wang, Lab Chip, 2012, 12, 1944 RSC.
Footnotes |
† Electronic supplementary information (ESI) available. See DOI: 10.1039/c4lc00596a |
‡ These authors have contributed equally to this work. |
|
This journal is © The Royal Society of Chemistry 2014 |
Click here to see how this site uses Cookies. View our privacy policy here.