DOI:
10.1039/C3MT00223C
(Paper)
Metallomics, 2014,
6, 77-81
A palladium label to monitor nanoparticle-assisted drug delivery of a photosensitizer into tumor spheroids by elemental bioimaging
Received
19th August 2013
, Accepted 15th November 2013
First published on 15th November 2013
Abstract
In this study, the cellular uptake of the second generation photosensitizer 5,10,15,20-tetrakis(3-hydroxyphenyl)porphyrin (mTHPP) was investigated using laser ablation coupled to inductively coupled plasma mass spectrometry (LA-ICP-MS) at a spatial resolution of 10 μm. To achieve high sensitivity, the photosensitizer was tagged with palladium. As a tumor model system, a 3D cell culture of the TKF-1 cell line was used. These tumor spheroids were incubated with the Pd-tagged photosensitizer embedded in poly(lactic-co-glycolic acid) (PLGA) nanoparticles to investigate the efficiency of nanoparticle based drug delivery. An accumulation of the drug in the first cell layers of the tumor spheroid was observed. In the case of nanoparticle based drug delivery, a significantly more homogeneous distribution of the photosensitizer was achieved, compared to tumor spheroids incubated with the dissolved photosensitizer without the nanoparticular drug delivery system. The infiltration depth of the Pd-tagged photosensitizer could not be increased with rising incubation time, which can be attributed to the adsorption of the photosensitizer onto cellular components.
Introduction
Photodynamic therapy has proven its value as a powerful alternative in the treatment of cancer and malignant tissue. The clinical application was first described by von Tappeiner and Jesionek as early as in 1903.1 Briefly, the therapy consists of two main procedures: the administration of a photosensitive drug (photosensitizer) and the subsequent illumination of the tumor.2 Here, the excitation of the photosensitizer with light of a specific wavelength leads to the generation of highly reactive oxygen species (e.g., singlet oxygen). These species are able to induce the programmed cell death (apoptosis) of the tumor cells. Photosensitizers can be classified into three generations, wherein first and second generation photosensitizers are used in clinical applications, while the third – target-specific photosensitizers – are still in the development phase.3 Hematoporphyrin derivatives (HpD) belong to the first generation. However, disadvantages such as the poor selectivity, the necessity of using significant concentrations of the drug to obtain a good efficiency and the high skin photosensitivity restrict the fields of application.4
The second generation consists of porphyrin derivatives, phthalocyanines, naphthalocyanines and chlorins (2,3-dihydroporphyrins). Advantages of these photosensitizers are a higher selectivity towards malignant tissue and the strong absorption peaks in the spectral region between 650 and 800 nm, which permit much better penetration of tissues.5 However, the strong hydrophobicity hinders the intravenous application, which calls for the development of appropriate delivery systems, e.g. the embedding of the photosensitizer into nanoparticles.6
The goal of the presented project is to develop a method to visualize the distribution of the photosensitizer in malignant tissue. While monitoring of the administrated drug can easily be performed by means of fluorescence microscopy,7,8 the approach is restricted to fluorescent compounds of interest. Therefore, we suggest elemental bioimaging based on the combination of laser ablation and inductively coupled plasma mass spectrometry (LA-ICP-MS) as an attractive alternative, which is characterized by excellent sensitivity and very low limits of detection. This technique was first used by Wang et al. for the spatially resolved analysis of biological tissues and is used increasingly for this purpose.9 Recently, several comprehensive reviews have been published concerning imaging of biological tissues using LA-ICP-MS.10–14
ICP-MS delivers best results for electropositive elements, which are characterized by a low natural abundance, thus resulting in low background signals. Therefore, C, H, O, and N as the constituents of the photosensitizer itself are not suitable for ICP-MS detection, and metal labeling is required. Lanthanides and platinum group metals are among the elements which meet the requirements for ICP-MS labels best. Therefore, the second generation photosensitizer 5,10,15,20-tetrakis(3-hydroxyphenyl)porphyrin (mTHPP),15,16 was tagged by complexation with palladium(II) as the central ion, which is shown in Fig. 1.17
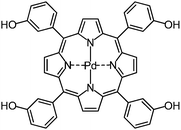 |
| Fig. 1 Structure of the photosensitizer [5,10,15,20-tetrakis(3-hydroxyphenyl)porphyrinato]-palladium(II) (mTHPP-Pd). | |
During the studies, the porphyrin complex was used in solution as the dissolved substance as well as in poly(lactic-co-glycolic acid) (PLGA) nanoparticles, in which the photosensitizer was embedded.8 Use of such colloidal drug carriers appears to be a promising strategy to enhance the delivery of pharmaceuticals into cells.
Materials and methods
General remarks
Dichloromethane (DCM), ethyl acetate (EtOAc) and methanol (MeOH) were purchased in p.a. quality. Palladium(II) acetate was purchased from Sigma-Aldrich. Products were purified by chromatography on silica gel (40–63 μm, Fluka, No. 60752, containing 0.1% Ca) and detection was carried out using a CAMAG variable UV detector (λ = 254/366 nm). The yield refers to the chromatographically purified product. The reaction was monitored using thin layer chromatography (TLC) analysis. NMR spectra were recorded on a Bruker AVIII 700 instrument. Chemical shifts are reported relative to tetramethylsilane (TMS, 1H: δ = 0.00 ppm) and the acetone-d6 solvent residual signal (1H: δ = 2.05 ppm; 13C: δ = 29.8 ppm). Coupling constants are given in Hz. The 13C spectrum is proton decoupled. Multiplicity is indicated as follows: s (singlet), d (doublet), dd (doublet of doublets), t (triplet), and br. s (broad singlet). The UV/Vis spectra were recorded on a SPECORD S300 VIS UV/Vis spectrometer (Analytik Jena, Jena, Germany) with acetone as solvent and quartz cuvettes of 1 cm path length. HRMS analysis was performed on an Agilent 6210 ESI-TOF instrument (Agilent Technologies, Santa Clara, CA, USA). The solvent flow rate was adjusted to 4 μL min−1 and the spray voltage was 4 kV. The drying gas flow rate was 15 psi (1 bar). All other parameters were adjusted for maximum abundance of the respective [M + H]+ ions. The melting point was determined using a Reichert Thermovar apparatus and is uncorrected. mTHPP was prepared according to known literature procedures15,16 and its spectroscopic data were compared with those reported in the literature.
Synthesis of mTHPP-Pd
The reaction was performed in a 100 mL round-bottom flask. Palladium(II) acetate (320 mg, 1.43 mmol) was suspended in MeOH (2 mL). To this suspension, a solution of mTHPP (300 mg, 0.44 mmol) in MeOH (15 mL) was added. Sodium acetate (20 mg, 0.24 mmol) was added and the resulting mixture was stirred for 12 h. The progress of the reaction was monitored by TLC (DCM–MeOH, 9
:
1, v/v). After this, the reaction mixture was transferred to a separatory funnel, diluted with water (200 mL) and extracted with EtOAc (250 mL). For better phase separation, brine (10 mL) was added. The phases were separated and the organic phase was washed with water (4 × 200 mL). The organic phase was dried with anhydrous Na2SO4. The drying agent was removed and the organic phase was evaporated to dryness. The remaining solid was taken up in a minimum amount of solvent (DCM–MeOH, 9
:
1, v/v) and applied to silica gel column chromatography for further purification using DCM–MeOH (9
:
1, v/v) as the eluent. mTHPP-Pd was isolated as the first fraction followed by brownish-colored non-fluorescent products (most probably porphyrin oxidation products). The fraction containing the product was evaporated to dryness, treated with DCM (in which it is insoluble) and suction filtered to obtain the pure product as a purple solid (281 mg, 0.36 mmol, 81%); m.p. > 300 °C. 1H NMR (700 MHz, acetone-d6): δ = 7.32 (dd, J = 8.3, 2.5 Hz, 4H, Ar), 7.60 (t, J = 7.8 Hz, 4H, Ar), 7.66 (d, J = 7.8 Hz, 4H, Ar), 7.71 (s, 4H, Ar), 8.93 (s, 8H, β-pyrrole-H), 8.94 ppm (br. s, 4H, OH). 13C NMR (176 MHz, acetone-d6): δ = 116.0, 122.4, 122.7, 126.7, 128.7, 131.9, 142.3, 143.6, 156.9 ppm. HRMS (ESI-TOF): m/z calc. for C44H29N4O4Pd [M + H]+: 782.1155, found: 782.1166. UV/Vis (acetone): λmax [log
ε (L mol−1 cm−1)] = 414.0 [5.54], 521.0 [4.47], 551.0 [3.49] nm.
Nanoparticle preparation
Embedding of mTHPP-Pd into nanoparticles was carried out using the emulsion diffusion method. PLGA solution (10% (w/v)) with mTHPP-Pd (1% (w/v)) in ethyl acetate was emulsified with 1% (w/w) polyvinyl alcohol (PVA) solution, using an Ultra Turrax (IKA, Staufen, Germany). Process parameters were conducted using a stirring speed of 21
000 rpm for 30 min. Finally, the dispersion was diluted with PVA solution and stirred for 16 h with 550 rpm to evaporate ethyl acetate. Using this method, nanoparticles with an average diameter of 250 nm and polydispersity index <0.1 were obtained, controlled by dynamic light scattering (Malvern Zetasizer Nano, Malvern, Herrenberg, Germany). The nanoparticles were purified by threefold centrifugation and redispersion in purified water (18
000g, 10 min).
Drug quantification was carried out using a HPLC–DAD system (Agilent Technologies 1200 series) with a Phenomenex Gemini C18 column (250 × 4.6 mm) and isocratic elution with 80% acetonitrile and 20% water with 0.1% trifluoroacetic acid (w/v). After dissolving the nanoparticles in acetone, mTHPP-Pd was quantified using the calibration curve of pure mTHPP-Pd. The detection wavelength was 413 nm.
Cell culture
TFK-1 cells were cultured in RPMI 1640 medium (Invitrogen, Karlsruhe, Germany) supplemented with 10% fetal bovine serum (FBS Gold, PAA, Pasching, Austria) and 50 U mL−1 penicillin and 50 μg mL−1 streptomycin (Invitrogen, Karlsruhe, Germany) in a 5% CO2 incubator at 37 °C and 85% humidity. The medium was changed every second day. Every week the cells were sub-cultured at a split ratio of 1 to 5 by treatment with 0.05% trypsin–EDTA.
Preparation of tumor spheroids
125
000 cells were seeded in a 125 mL Spinner Flask and cultured for 15 days in a 5% CO2 incubator at 37 °C and 85% humidity at a stirring rate of 75 rpm (magnet stirrer: 2 mag, Munich, Germany). The medium was changed every third day in the first week, and every second day in the second week of culturing.
Preparation of samples for uptake studies
15 day old tumor spheroids were used for incubation with mTHPP. Therefore, spheroids were transferred into 6 well ultra-low-attachment cell culture plates (Corning, Amsterdam, The Netherlands) and incubated with medium containing 0.8 μg mL−1 or 40.7 μg mL−1mTHPP-Pd either incorporated into PLGA-nanoparticles or as the dissolved substance. After an incubation time of 24 h or 48 h, tumor spheroids were collected and washed twofold with PBS (pH 7.2; Invitrogen, Karlsruhe, Germany) to remove substance that was not taken up. Embedding of the spheroids in Tissue-Tek® O.C.T.™ (Sakura, Staufen, Germany) was followed by cutting the samples into 5 μm thin sections using a cryostat (Leica CM 3050, Leica, Nussloch, Germany). Sections were mounted on SuperFrost Ultra Plus™ glass slides (Menzel, Braunschweig, Germany). All procedures in the presence of a photosensitizer were performed under minimal light conditions to avoid activation of the compound.
Imaging of tumor spheroid sections
LA-ICP-MS experiments were performed using a commercial laser ablation system using a frequency quintupled Nd:YAG laser (LSX 213, CETAC Technologies, Omaha, NE, USA) with a wavelength of 213 nm, which was coupled to a quadrupole-based inductively coupled plasma mass spectrometer (iCAP Qc, Thermo Fischer Scientific, Bremen, Germany) by means of a transfer line. The tumor spheroids were ablated in a line by line scan with 70% of the maximum laser energy, 20 Hz laser shot frequency, 10 μm spot diameter and 10 μm s−1 scan speed. The obtained dry aerosol was transported to the ICP torch with an Ar–He gas mixture. Additionally, a ruthenium solution as the internal standard with a concentration of 10 ng L−1 was simultaneously introduced by a PFA nebulizer and a cyclonic spray chamber. For maximum sensitivity and to ensure low levels of oxides, the ICP-MS was tuned on a daily basis. Possible interferences of e.g. [40Ar + 64Cu]+ were minimized by utilization of a collision/reaction cell with a He–H2 gas mixture in kinetic energy discrimination (KED) mode. The isotopes 105Pd, 106Pd, and 103Rh were detected with dwell times of 0.5 s, 0.4 s and 0.1 s, respectively. The recorded data were evaluated using a home-built software package.
Results and discussion
To investigate the cellular uptake and intracellular accumulation of the photosensitizer in tumor tissue, the bile duct carcinoma cell line TFK-1 was used18 and a 3D culture of these cells was performed. The formation of the TFK-1 tumor spheroids was induced by the Spinner flask culture method. The spheroids had a size range between 200 μm and 500 μm. Due to the application of tumor spheroids, the number of animal experiments can be decreased and the use of cell cultures allows for an easier handling and more reproducible experimental conditions. Furthermore, the 3D structure gives a more realistic model compared to monolayer or suspension cell cultures and therefore improves comparability to in vivo experiments.
To demonstrate the cellular uptake and intracellular accumulation of mTHPP-Pd, tumor spheroids were incubated for 24 h or 48 h with different concentrations (0.8 μg mL−1, 8.2 μg mL−1, and 40.7 μg mL−1) of the photosensitizer either in solution or entrapped in PLGA nanoparticles. After incubation, the spheroids were washed with phosphate buffered saline, embedded in Tissue-Tek® O.C.T.™ and cryosectioned into 5 μm thin slices. The investigation of the distribution of mTHPP-Pd using fluorescence microscopy revealed that this method is not sufficiently sensitive to detect the photosensitizer entrapped in PLGA nanoparticles in the relevant concentration range (data not shown). Hence, the distribution of the photosensitizer was analyzed using a laser ablation system coupled to an ICP-MS, where the isotopes 105Pd, 106Pd and 103Rh were detected. A ruthenium solution was nebulized as the internal standard and the generated wet aerosol was mixed with the stream of the dry aerosol coming from the laser ablation unit for examination and stabilization of the plasma conditions. Images were generated by a line by line scan with a lateral resolution of 10 μm.
Fig. 2 shows the images obtained for the tumor spheroids incubated for 48 h with 8.2 μg mL−1 [(a) + (b)] and 40.7 μg mL−1 [(c) + (d)] with mTHPP-Pd loaded PLGA nanoparticles. It is obvious that an enrichment of the drug occurs mainly within the first cell layers of the spheroid. This can be attributed to the very high affinity of the low polarity compounds to proteins.
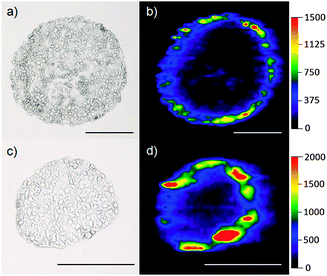 |
| Fig. 2 Images of TFK-1 tumor spheroid sections incubated with mTHPP-Pd PLGA nanoparticles for 48 h [(a) + (b) 8.2 μg mL−1, (c) + (d) 40.7 μg mL−1]. The scale represents a length of 200 μm in all images. The 105Pd signal is used for data evaluation. Intensity is stated in counts per second (cps). Images were received using a laser spot diameter of 10 μm. | |
The two spheroids presented in Fig. 2 have been selected to represent one of the largest [(a) and (b), diameter approximately 470 μm] and one of the smallest spheroid diameters [(c) and (d), diameter approximately 250 μm] found in this cell culture. Although the spheroids differ in their diameter, the depth of penetration of the photosensitizer is similar on an absolute scale. The detected intensities of the Pd signal increase with rising concentration of the photosensitizer.
Images of tumor spheroids incubated with 0.8 μg mL−1 and 40.7 μg mL−1mTHPP-Pd for 24 h embedded in nanoparticles and as the dissolved substance are presented in Fig. 3. Differences in intensities between (b) and (d) represent the two concentrations of the incubated mTHPP-Pd. Again, a similar penetration depth of the photosensitizer is observed. In the case of the tumor spheroid incubated with the dissolved substance [(e) + (f)], a strong accumulation of the drug in specific areas is visible. Additionally, spheroids without detectable Pd intensities were located. This unspecific accumulation of the photosensitizer can be explained by the hydrophobic character of the drug. Due to changes of the chemical environment by infiltration of the drug into the tumor, the drug precipitates. Even in the optical microscopic images, this accumulation is observed as red spots of the Pd complex. Their position correlates well with the highest Pd signals located in the mass spectrometric image. In the case of nanoparticles, however, the polymer PLGA keeps the photosensitizer in solution and a much more even distribution of the complex in the outer parts of the spheroid is observed.
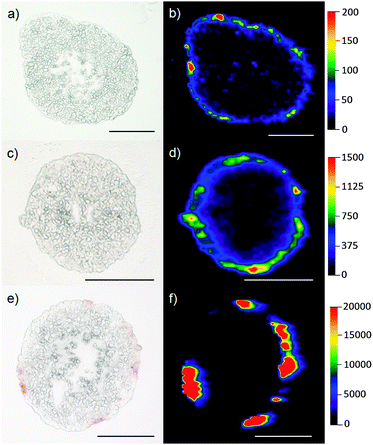 |
| Fig. 3 Images of TFK-1 tumor spheroid sections incubated with mTHPP-Pd PLGA nanoparticles for 24 h [(a) + (b) 0.8 μg mL−1, (c) + (d) 40.7 μg mL−1] and the dissolved substance [(e) + (f) 40.7 μg mL−1]. The scale represents a length of 200 μm in all images. The 105Pd signal is used for data evaluation. Intensity is stated in counts per second (cps). Images were received with a laser spot diameter of 10 μm. | |
Conclusions
These data unambiguously confirm that nanoparticle-assisted drug delivery is an extremely powerful approach for the delivery of low polarity pharmaceuticals into cells.
This work proves that the metal labeling approach presented for photosensitizers is a highly promising model to study the penetration of drugs or other xenobiotics into cultured cells. Future work should be directed towards multielement labeling of several compounds of interest with simultaneous multielemental bioimaging.
Acknowledgements
Parts of this study were supported by the Cells in Motion Cluster of Excellence (CiM-EXC 1003), Münster, Germany (project FF-2013-17).
References
- H. von Tappeiner and H. Jesionek, Muench. Med. Wochenschr., 1903, 47, 2042–2044 Search PubMed.
- M. Triesscheijn, P. Baas, J. H. M. Schellens and F. A. Stewart, Oncologist, 2006, 11, 1034–1044 CrossRef CAS PubMed.
- M. O. Senge and J. C. Brandt, Photochem. Photobiol., 2011, 87, 1240–1296 CrossRef CAS PubMed.
- C. Hopper, Lancet Oncol., 2000, 1, 212–219 CrossRef CAS.
- Y. N. Konan, R. Gurny and E. Allémann, J. Photochem. Photobiol., B, 2002, 66, 89–106 CrossRef CAS.
- D. K. Chatterjee, L. S. Fong and Y. Zhang, Adv. Drug Delivery Rev., 2008, 60, 1627–1637 CrossRef CAS PubMed.
- H. Ding, R. Mora, J. Gao and B. D. Sumer, Otolaryngol.–Head Neck Surg., 2011, 145, 612–617 CrossRef PubMed.
- K. Löw, T. Knobloch, S. Wagner, A. Wiehe, A. Engel, K. Langer and H. von Briesen, Nanotechnology, 2011, 22, 245102–245113 CrossRef PubMed.
- S. Wang, R. Brown and D. J. Gray, Appl. Spectrosc., 1994, 48, 1321–1325 CrossRef CAS.
- J. S. Becker and J. S. Becker, Biomed. Spectrosc. Imaging, 2012, 1, 187–204 CAS.
- J. S. Becker, M. Zoriy, A. Matusch, B. Wu, D. Salber, C. Palm and J. S. Becker, Mass Spectrom. Rev., 2010, 29, 156–175 CAS.
- M. Careri and A. Mangia, Anal. Bioanal. Chem., 2011, 399, 2585–2595 CrossRef CAS PubMed.
- I. Konz, B. Fernández, M. L. Fernández, R. Pereiro and A. Sanz-Medel, Anal. Bioanal. Chem., 2012, 403, 2113–2125 CrossRef CAS PubMed.
- D. Hare, C. Austin and P. Doble, Analyst, 2012, 137, 1527–1537 RSC.
- A. Wiehe, Y. M. Shaker, J. C. Brandt, S. Mebs and M. O. Senge, Tetrahedron, 2005, 61, 5535–5564 CrossRef CAS PubMed.
- S. A. Syrbu and A. S. Semeikin, Russ. J. Org. Chem., 1999, 35, 1236–1239 CAS.
- A. Wiehe, H. Stollberg, S. Runge, A. Paul, M. O. Senge and B. Röder, J. Porphyrins Phthalocyanines, 2001, 5, 853–860 CrossRef CAS.
- S. Saijyo, T. Kudo, M. Suzuki, Y. Karayose, M. Shinoda, T. Muto, K. Fukuhara, T. Suzuki and S. Matuso, Tohoku J. Exp. Med., 1995, 177, 61–71 CrossRef CAS.
|
This journal is © The Royal Society of Chemistry 2014 |
Click here to see how this site uses Cookies. View our privacy policy here.