DOI:
10.1039/C3RA45809A
(Communication)
RSC Adv., 2014,
4, 379-385
A microfluidic chip for ammonium sensing incorporating ion-selective membranes formed by surface tension forces
Received
14th October 2013
, Accepted 6th November 2013
First published on
7th November 2013
Abstract
A microfluidic chip incorporating a series of polymeric ion-selective membranes (ISMs) is proposed for the detection of ammonium ions. In the proposed approach, the ISMs are formed by means of a simple flow-through forming technique, in which a polymer liquid doped with ammonium ionophores is injected into the microchip and attached to a series of diamond-like microstructures patterned along the center of the microchannel under the effects of surface tension forces and two neighboring sheath flows. The experimental results show that the proposed microsensor exhibits a linear response when detecting ammonium ions with a concentration of 10−4 to 103 ppm (R2 ∼ 0.9689). Furthermore, the detection limit is shown to be as low as 0.1 ppb. Finally, the time response when measuring 0.01 ppm of ammonium ions is just 4 s (95% standard), which is far faster than that of typical commercial ISE products (∼minutes) Overall, the results presented in this study show that the proposed microchip provides a low-cost yet highly-sensitive means of performing the continuous detection of ammonium ions.
I. Introduction
The electrochemical detection of redox reactions is one of the most promising techniques for analyzing specific ions in solution.1,2 In such techniques, the detection process is generally performed by permeating the sample fluid through an ion selective membrane and then measuring the resulting change in the membrane potential.3 However, the success of such methods relies on there being a clearly discernible difference between the redox potential of the target ions and that of the other ions in the sample fluid. To overcome this problem, ion selective electrodes (ISEs) have been proposed for filtering out the undesired ions such that only the target ions remain for electrochemical detection.4 ISEs typically comprise a standard electrochemical electrode coated with an ion-selective membrane for detecting specific ion samples in solutions. This technique has have been widely applied in the food, medical research, and environmental monitoring fields.
The first ISE was proposed by Ross et al.5 as far back as 1967, and incorporated a liquid ion-exchanger for the detection of calcium ions. Inspired by the first work, the ammonium ISE was then demonstrated utilizing ammonium specific glass membrane.6 ISE membranes can be fabricated using a variety of materials, including solids, organic liquids and polymeric films. However, polyvinyl chloride (PVC) membranes have attracted particular attention due to their permeability, plasticity and ease of fabrication.7 For example, Bloch et al.8 fabricated ISE membranes for calcium ion detection based on a PVC matrix doped with tributyl phosphate or tributyl phosphate plus thenoyltrifluoroacetone. A number of reports have then reported to confirm the feasibility of ion detection using ionophores doped polymer films. Schaller et al.9 investigated the effects of different ionic additives on the behaviors of cation- and anion-selective electrodes with PVC matrices. In addition, the selectivity coefficients of various the devices with the PVC-based ISEs were experimentally evaluated.10,11 Alternatively, PVC matrix doped with calcium ionophores was used to measure the calcium concentration in blood serum. Specific metal ions including copper(II) ion,12 Ce(III)13 ion were also experimentally inspected using the corresponding ion selective membranes. Bakker14 presented a method for improving the selectivity of PVC-based ISEs by conditioning the electrode membrane in a solution of a discriminated ion equal to the cation of the tetraphenylborate salt incorporated in the membrane. Ding et al.15 presented a polymeric membrane ion selective electrode for potentiometric sensing by utilizing the external current to control the diffusion of the ions accurately. In general, PVC-based ion-selective membranes are characterized by a short response time and a long lifetime. As a result, they have significant potential for the development of sensors for the detection of common analytes such as ammonium, hydrogen, sodium, and so on.
Advances in the micro-electro-mechanical systems (MEMS) technology field have resulted in the development of many microfluidic devices for such diverse tasks as cell sorting and counting, polymerase chain reaction (PCR), species mixing, environmental monitoring, and so on. A number of works have been reported which used miniaturized microchips with planar electrodes for electrochemical detection of bio-samples.16,17 In addition, microfluidic devices incorporating polymeric or liquid membrane electrodes for ion-selective detection have attracted particular attention in recent years.18 For example, Berduque et al.19 presented a microfluidic chip incorporating an electrochemically-modulated liquid vertical bar for the extraction of specific analyte ions. Lim et al.20 fabricated a microfluidic chip incorporating a tetraphenylphosphonium (TPP+) selective microelectrode sensor for evaluating the concentration of isolated mitochondria (Heb7A). Tantra and Manz18 presented a microfluidic sensor based on a single ISE membrane for the continuous detection of barium ions. However, the device had a relatively poor detection performance due to the large membrane thickness (∼500 μm). Hisamoto et al.21 developed microfluidic sensors incorporating single and parallel dual-membrane structures prepared using organic/aqueous two-layer flows and organic/aqueous/organic three-layer flows, respectively. However, the fabrication process relies on a precise control of the various reactive solutions in order to ensure the stable and continuous formation of the membrane.
Ammonium is widely used throughout the plastics, textiles and pharmaceutical fields and is one of the most common industrial coolants. However, ammonium is associated with many health risks, including irritation of the nose and throat, coughing, shortness of breath, tightness in the chest, and even death. As a result, accurate methods are required for detecting the concentration of ammonium in order to safeguard human health.22 Accordingly, ammonium ion selective electrode was mainly applied to detect the ammonium ions produced by the enzymatic reaction of urea,23 creatinine24 or amino acids.25–27 The present study proposes a highly-sensitive microfluidic chip for ammonium detection incorporating a series of polymeric ion-selective membranes (ISMs) with the ammonium ionophore. In fabricating the proposed device, the ISMs are formed using a simple flow-through technique, in which a polymer liquid doped with ammonium ionophores is injected into the microchip and is then self-attached to a series of diamond-like microstructures distributed along the center of the microchannel under the effects of surface tension forces and two neighboring sheath flows. The performance of the proposed device is evaluated by means of cyclic voltammetry tests using NH4Cl solutions with ammonium concentrations ranging from 10−4 to 103 ppm. In general, the results show that the thin characteristic width of the individual ISMs and the large overall diffusion area provided by the multiple ISMs within the microchannel lead to both a low detection limit and a rapid response time.
II. Materials and methods
A. Design concept
Fig. 1 illustrates the basic principle of the flow-through forming technique used in this study to fabricate the ISMs within the microfluidic sensor. As shown, the center of the microchannel is patterned with a series of diamond-like microstructures with a side-length of 50 μm and a pitch of 200 μm. A PVC solution doped with ammonium ionophores is injected into the channel and a vacuum suction force is then applied at the channel outlet; prompting the formation of two air sheath flows on either side of the polymer flow. The combined effects of surface tension forces and the neighboring sheath flows prompt the formation of PVC membranes between each pair of neighboring microstructures. In practice, the membrane thickness depends on various factors, including the dimensions of the diamond-like microstructures, the viscosity of the PVC solution, the material properties of the microchannel, and the magnitude of the vacuum suction force. In the present study, the PVC membranes were found to have a thickness of less than 50 μm. A series of ion selective membranes with uniform thickness can be formed in the micro-channel with this simple approach.
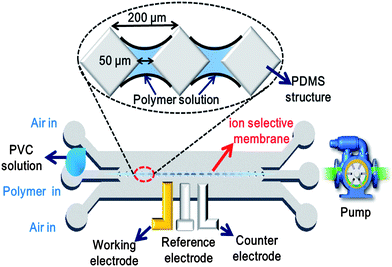 |
| Fig. 1 Schematic illustration showing formation of ISMs under effects of surface tension forces and neighboring sheath flows. | |
B. Microchip fabrication
Fig. 2 illustrates the basic steps in the microfluidic sensor fabrication process. As shown, the microfluidic chip comprised two layers, namely a PDMS layer incorporating the microchannels and a glass substrate (26 mm × 76 mm) containing the counter, reference and working electrodes. The details for fabricating the microchannels can be found in the previous report.28 The fabrication process commenced by patterning the microfluidic channels using a standard PDMS replication technique (Fig. 2(A)) Using a vinyl mask patterned with cutouts corresponding to the three electrodes (2 mm in width, 2 mm in pitch), a sputtering process was performed to deposit a chromium (Cr) seed layer with a thickness of 170 nm on the surface of the glass substrate. Further sputtering operations were then performed to deposit platinum (Pt), silver (Ag) and gold (Au) films with thicknesses of 600 nm, 1 μm and 660 nm as the counter electrode, reference electrode and working electrode, respectively (Fig. 2(B)). The silver electrode was then chlorinated to form a Ag/AgCl reference electrode by immersing the patterned substrate in 0.1 M HCl solution and applying a constant electric potential of 1.5 V for 10 s (Fig. 2(C)). The PDMS replica was drilled with via holes and carefully aligned with the glass substrate. The replica and substrate were treated with oxygen plasma and were then bonded together to form a sealed microfluidic channel. (Fig. 2(D) and (E)) Finally, the PVC solution was injected into the microchannel and the membranes formed at room temperature for 5 min using the method described in the previous section (Fig. 2(F)).
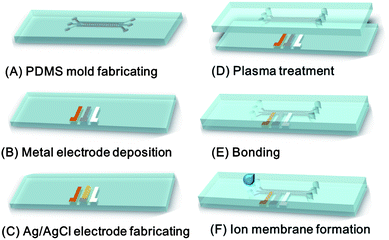 |
| Fig. 2 Schematic overview of fabrication process used to realize microfluidic chip with integrated ISMs. | |
Fig. 3(A) and (B) present a photograph of the fully-assembled ISM microchip and an SEM image of the PVC membrane, respectively. It is seen in Fig. 3(A) that the chip comprises a central microchannel (dimensions: 150 × 150 μm2) containing the patterned diamond-like microstructures and PVC membranes and two outer microchannels (dimensions 15 mm × 150 μm) for the sample solution and electrolyte solution, respectively. It is also noted that the bonding process results in the formation of sealed microfluidic channels without clogging. The SEM image presented in Fig. 3(B) shows that the formed PVC membrane has a rough surface, and therefore facilitates the diffusion of the ammonium ions from the sample channel to the electrochemical detection channel. In addition, it is observed that a good contact exists between the PVC membrane and the adjacent PDMS microstructure.
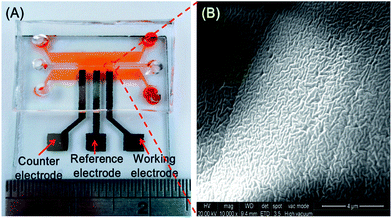 |
| Fig. 3 (A) Photograph of assembled microfluidic chip. (B) SEM image of membrane surface (10 000×). | |
C. Reagents and solutions
PVC, dibutyl sebacate and ammonium ionophores were purchased from Fluka (Buchs, Switzerland). Sodium chloride and ammonium chloride were purchased from Showa Chemical Industry Co. Ltd. (Tokyo, Japan). THF (tetrahydrofuran) was obtained from Alfa Aesar (Karlsruhe, Germany). All of the chemical reagents and solvents were of an analytical grade. Moreover, all of the reagents were prepared using deionized (DI) water. Finally, all of the tests were performed using 15 mM NaCl as the electrolyte solution.
D. Preparation of polymer material
In accordance with the standard procedure prescribed in the Fluka catalog, the polymer solution used to fabricate the PVC membranes was prepared by dissolving 33 wt% PVC, 66 wt% dibutyl sebacate and 1 wt% ammonium ionophores in 5 ml THF. The mixture was then injected into the microchannel in order to form PVC membranes using the method described in Section B.
E. Experimental setup
Fig. 4 presents a schematic illustration of the experimental setup used to evaluate the sensing performance of the microfluidic sensor. The electrochemical analysis procedure was performed using a CHI 611a Electrochemical Analyzer (CH Instruments Inc., USA). Before conducting electrochemical detection, the microchip was flushed with DI water in order to wet the membranes. 10 μL of sample solution and 10 μL of electrolyte were then injected into the microchip by means of syringe pumps. As the sample flowed through the ISM contact area, ammonium ions diffused through the doped PVC membranes into the electrolyte channel and were electrochemically detected by the ion-selective electrode (ISE). Having completed the detection process, the microchip was flushed once again before introducing the next sample.
 |
| Fig. 4 Schematic illustration of experimental setup used to characterize microfluidic sensor. | |
F. Electrochemical detection
According to the developed microfluidic chip is using the electrochemical method for detect the sample concentration variation. The chip is design for including a working electrode, a counter electrode and a reference electrode, which the three-electrode system is using to detect the redox signals of the sample solution by the cyclic voltammetry approach. Since the ammonium chloride dissolved in the water was completely dissociated, such the ammonium ions can transport through the sensing membrane along the sample flow. It is noted that the three electrochemical electrodes were designed at the same side to the ion selective membrane for EC measurements. Although some ISE detection mechanism used two electrodes on the different sides of the ion selective membrane, this approach is difficult to be used for long-term measurement since the electrode in the sample solution size may be contaminated or corroded by the sample solution. In this regards, it is of great important to design the three electrodes at the other side of the ion selective membrane.
G. Electrode testing
The experiments commenced by evaluating the performance of the ISE in the absence of an ion-selective membrane. Fig. 5(A) presents the corresponding cyclic voltammetry results obtained using NH4Cl solutions with ammonium ion concentrations ranging from 101 ppm to 103 ppm. It is seen that for low ammonium concentrations (i.e., 101 and 102 ppm), there is virtually no change in the detection current as the potential is first ramped and then inverted. Moreover, the current responses of the two samples are almost indistinguishable from one another. Furthermore, for a high ammonium concentration (i.e., 103 ppm), the current response exhibits significant instability.
 |
| Fig. 5 (A) Cyclic voltammetry results obtained without ISM for NH4Cl solutions with different ammonium concentrations. (B) Cyclic voltammetry results obtained using ISM attached directly to electrolyte reservoir for NH4Cl solutions with different ammonium concentrations. | |
In a traditional electrochemical detection process, a PVC membrane is fabricated by evaporating a solution of PVC and THF on a clean glass substrate. The solidified membrane is then stripped from the glass substrate and attached to the top of an electrolyte reservoir using THF. The sample solution is then dropped onto the membrane and the ions allowed to permeate through the membrane into the electrolyte. The target ion concentration is then determined by measuring the corresponding change in the current at the working electrode. Fig. 5(B) presents the cyclic voltammetry results obtained using this method for NH4Cl solutions with ammonium ion concentrations ranging from 10−2 to 103. It is seen that the current response is clearly differentiated for the samples with different ammonium concentrations. Furthermore, for higher ammonium concentrations, the current response is far more stable than that in Fig. 5(A). Thus, the effectiveness of the PVC membrane in filtering out the undesired ions in the sample solution is confirmed. However, the sensor requires a few minutes to attain equilibrium conditions. Furthermore, the process of attaching the membrane to the electrolyte reservoir is inconvenient and time-consuming.
III. Results and discussions
Fig. 6(A) presents a cross-sectional SEM image of the ion-channel containing the diamond-like microstructures and PVC membranes. Meanwhile, Fig. 6(B) presents a close-up SEM image of a single PVC membrane and PDMS microstructure. In general, the images confirm the success of the proposed flow-through fabrication method in realizing PVC membranes with a thin (∼50 μm) and uniform thickness along the center of the ion channel by means of surface tension forces and the constraining effect of the neighboring sheath flows. The sensing performance of the produced ISE microfluidic device was investigated by cyclic voltammetry measurement of ammonium ion solutions of different concentrations. Fig. 7 shows the cyclic voltammetry results obtained using the proposed ISM microfluidic sensor for NH4Cl samples containing ammonium concentrations ranging from 10−2 to 103 ppm. Note that the scanning rate is set as 0.1 V s−1 in order to maximize the response current. The CV measurement was to confirm the capability of the produced EC electrodes inside the microfluidic channel. Results showed that the EC electrodes in the microfluidic channel provided a nice sensing performance on detecting ammonium ions. It is seen that the current responses obtained for the samples with different ammonium concentrations are clearly distinguishable from one another. As a result, the ability of the proposed sensor to perform ammonium detection over a wide concentration range is confirmed. As expected, the measured current response increases with an increasing ammonium concentration; indicating that the membranes have sufficient porosity to permit the rapid diffusion of even large numbers of ammonium ions. Finally, significant peaks are observed in the current response at an applied potential of ±0.28 V for all values of the ammonium concentration. As a result, the stability of the redox reaction at the ISE is confirmed.
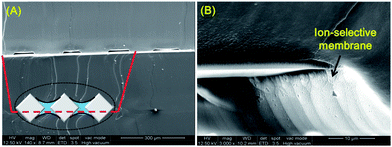 |
| Fig. 6 (A) Cross-sectional SEM image of ion-channel structure. Note that the dash line indicates the cross-section where the SEM was took. (B) SEM image of ISM within ion-channel. | |
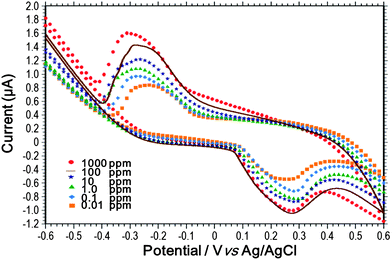 |
| Fig. 7 Cyclic voltammetry results obtained using ISM microfluidic sensor for NH4Cl solutions with different ammonium concentrations. | |
Fig. 8 illustrates the variation of the measured current response with the ammonium concentration over the range of 10−4 to 103 ppm. Note that the error bars indicate the variation in the current signal as obtained over 4 separate CV measurements, as shown in Fig. 7, at the same ammonium concentration. It is seen that the proposed ISM microchip has good linearity (R2 = 0.9689) over the considered range. In addition, the small magnitude of the error bars indicates that the proposed sensor has good reproducibility at each of the considered concentration values. Notably, the detection range of the proposed sensor is far greater than that of typical commercial ISE sensors (10−1 to 104 ppm). In particular, the minimum detection limit of the proposed sensor, i.e., 0.1 ppb, is significantly lower than that of most commercial devices, i.e., 0.1 ppm. In other words, the results confirm that the thinner thickness of the individual PVC membranes and the greater overall diffusion area provided by the multiple ISMs within the microchannel result in a significantly improved sensing performance. The ammonium ions permeated to the ion selective membrane under the condition of a constant temperature, the current change is positively correlated to the analyte concentration. Therefore, the nice linearity confirmed that high performance electrochemical detection can be achieved using the ISE microfluidic system from very small sample volumes.
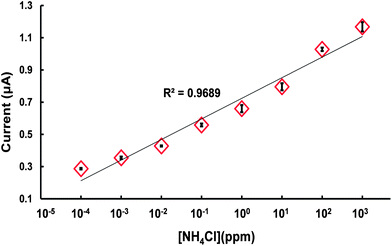 |
| Fig. 8 Current response of ISM microfluidic sensor for NH4Cl solutions with different ammonium concentrations. | |
Actually, even though the sensor is most selective to ammonium, all ammonium ion-selective electrodes suffer interference from other ions especially for sodium and potassium. In this regards, two major “non-target ions” of sodium and potassium ions were used for the ion interference test. The interference ions would induce the current response at high concentration levels. Some literatures reported that potassium ion of 23 mg l−1 and 821 mg l−1 of sodium ion could induce the current response equivalent to about 1 mg l−1 of ammonium ion to an ammonium ISE.29,30 To evaluate the selectivity of the proposed sensor, a series of sensing experiments were performed using five different samples, namely NH4Cl, KCl, NaBr, NH4Cl
:
KCl (9
:
1) and NH4Cl
:
NaBr (9
:
1). Fig. 9 presents the corresponding results. It is seen that for the pure KCl and NaBr samples, the current response has a low value; particularly for sample concentrations of 101, or less. Furthermore, it is observed that when Na+ and K+ ions are added to the NH4Cl solution, the current response is lower than that for the pure NH4Cl solution. Thus, the results confirm the highly selective properties of the membranes and electrochemical detection electrode within the proposed sensor.
 |
| Fig. 9 Comparison of NH4Cl sample solution on Na+ and K+ interferences in five different matrices. | |
Fig. 10 presents the time response of the proposed ISM microchip when detecting NH4Cl solutions containing ion concentrations ranging from 10−2 to 1.0 ppm. Note that the microchip was flushed thoroughly using the new sample following each test run. As expected, the results show that the time required to reach 95% of the final current response increases with a reducing ammonium concentration. However, in every case, the measured current response reaches 95% of the equilibrium value in less than 4 s. It is noted that this response time is significantly faster than that of commercial ISE devices, which typically have a response time of several tens of seconds or even minutes.
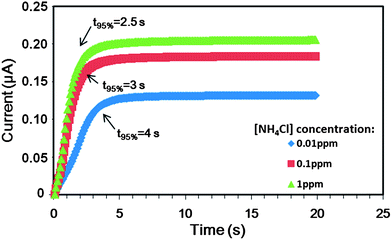 |
| Fig. 10 Time response of ISM sensor while sensing NH4Cl solutions with different ammonium concentrations using the thinner ion selective membrane. | |
IV. Conclusions
This study has presented a low-cost microfluidic sensor incorporating multiple ionophore-doped PVC ion-selective membranes (ISMs) for the detection of ammonium ions. The ISMs are fabricated by means of a simple flow through technique and permit a significant increase in the diffusion area within the ion-channel. As a result, the detection performance of the proposed device is greatly improved compared to that of a traditional ISE device with a single membrane. Furthermore, the membrane thickness of each ISM is less than 50 μm, and thus the response time and sensitivity of the proposed device are further improved. The experimental results have shown that the sensor has good linearity (R2 = 0.9689) for ion concentrations in the range of 10−4 to 103 ppm. Moreover, the proposed device has a minimum detection limit of 0.1 ppb, which is significantly lower than that of most commercial devices (∼0.1 ppm). It has been shown that the proposed device has high selectivity for ammonium ions and successfully suppresses the interference caused by undesired ions (e.g., Na+ and K+). Finally, it has been shown that the sensor has a response time of less than 4 s (95% standard) when applied to the detection of NH4Cl solutions with ion concentrations ranging from 10−3 to 10−1 ppm. It is noted that this response time is significantly shorter than that of commercial ISE devices, which typically have a response time of several tens of seconds or even minutes. Overall, the results confirm that the proposed microchip provides a low-cost yet highly-sensitive means of performing the continuous detection of ammonium ions. The present study has considered the specific case of ammonium sensing, and has doped the PVC membranes accordingly. However, the method proposed in this study for fabricating the ISMs can be easily extended to the realization of sensors for different analytes simply by changing the enzyme used to dope the PVC membrane.
References
- Y.-C. Wei, L.-M. Fu and C.-H. Lin, Microfluid. Nanofluid., 2013, 14, 583–590 CrossRef CAS.
- C.-M. Chen, G.-L. Chang and C.-H. Lin, J. Chromatogr., A, 2008, 1194, 231–236 CrossRef CAS PubMed.
- T. Guinovart, M. Parrilla, G. A. Crespo, F. X. Rius and F. J. Andrade, Analyst, 2013, 138, 5208–5215 RSC.
-
T.-Y. Chiang, L.-M. Fu, C.-H. Tsai and C.-H. Lin, Proceedings MicroTAS '12 Conference, 2012.
- J. W. Ross, Science, 1967, 156, 1378–1379 CrossRef CAS.
- G. Goodfellow and H. Webber, Analyst, 1972, 97, 95–103 RSC.
- S. Amemiya, Y. Kim, R. Ishimatsu and B. Kabagambe, Anal. Bioanal. Chem., 2011, 399, 571–579 CrossRef CAS PubMed.
- R. Bloch, A. Shatkay and H. A. Saroff, Biophys. J., 1967, 7, 865–877 CrossRef CAS.
- U. Schaller, E. Bakker, U. E. Spichiger and E. Pretsch, Anal. Chem., 1994, 66, 391–398 CrossRef CAS.
- G. J. Moody and J. D. R. Thomas, Talanta, 1971, 18, 1251–1252 CrossRef CAS.
- A. Craggs, G. J. Moody and J. D. R. Thomas, J. Chem. Educ., 1974, 51, 541–544 CrossRef CAS.
- I. M. Isa, M. I. Saidin, M. Ahmad, S. Mustaffa and S. A. Ghani, Int. J. Electrochem. Sci., 2012, 7, 9526–9536 CAS.
- H. A. Zamani, M. R. Ganjali and M. Adib, Sens. Actuators, B, 2007, 120, 545–550 CrossRef CAS PubMed.
- E. Bakker, J. Electrochem. Soc., 1996, 143, L83–L85 CrossRef CAS PubMed.
- J. Ding and W. Qin, J. Am. Chem. Soc., 2009, 131, 14640–14641 CrossRef CAS PubMed.
- J. Wang, M. P. Chatrathi, B. M. Tian and R. Polsky, Anal. Chem., 2000, 72, 2514–2518 CrossRef CAS.
- K. T. Liao, C. M. Chen, H. J. Huang and C. H. Lin, J. Chromatogr., A, 2007, 1165, 213–218 CrossRef CAS PubMed.
- R. Tantra and A. Manz, Anal. Chem., 2000, 72, 2875–2878 CrossRef CAS.
- A. Berduque, J. O'Brien, J. Alderman and D. W. M. Arrigan, Electrochem. Commun., 2008, 10, 20–24 CrossRef CAS PubMed.
- T. S. Lim, A. Davila, D. C. Wallace and P. Burke, Lab Chip, 2010, 10, 1683–1688 RSC.
- H. Hisamoto, Y. Shimizu, K. Uchiyama, M. Tokeshi, Y. Kikutani, A. Hibara and T. Kitamori, Anal. Chem., 2003, 75, 350–354 CrossRef CAS.
- K. Yasuda, H. Miyagi, Y. Hamada and Y. Takata, Analyst, 1984, 109, 61–64 RSC.
- A. Senillou, N. Jaffrezic-Renault, C. Martelet and S. Cosnier, Talanta, 1999, 50, 219–226 CrossRef CAS.
- M. Altikatoglu, E. Karakus, V. Erci, S. Pekyardimci and I. Isildak, Artif. Cells, Blood Substitutes, Biotechnol., 2012, 1–6 Search PubMed.
- M.-J. Syu and Y.-S. Chang, Biosens. Bioelectron., 2009, 24, 2671–2677 CrossRef CAS PubMed.
- M. Ghauri and J. Thomas, Analyst, 1994, 119, 2323–2326 RSC.
- K. Suzuki, D. Siswanta, T. Otsuka, T. Amano, T. Ikeda, H. Hisamoto, R. Yoshihara and S. Ohba, Anal. Chem., 2000, 72, 2200–2205 CrossRef CAS.
- C. H. Lin, G. B. Lee, Y. H. Lin and G. L. Chang, J. Micromech. Microeng., 2001, 11, 726–732 CrossRef CAS.
- M. Cortina-Puig, X. Munoz-Berbel, M. del Valle, F. J. Munoz and M. A. Alonso-Lomillo, Anal. Chim. Acta, 2007, 597, 231–237 CrossRef CAS PubMed.
- H. D. Shen, T. J. Cardwell and R. W. Cattrall, Analyst, 1997, 122, 89–93 RSC.
|
This journal is © The Royal Society of Chemistry 2014 |
Click here to see how this site uses Cookies. View our privacy policy here.