DOI:
10.1039/C3RA46208K
(Paper)
RSC Adv., 2014,
4, 12415-12421
Wavelength and shape dependent SERS study to develop ultrasensitive nanotags for imaging of cancer cells†
Received
29th October 2013
, Accepted 2nd January 2014
First published on 6th January 2014
Abstract
The Raman signal enhancement depends mainly on four critical factors. These factors are localized surface plasmon resonance (LSPR) of gold substrates along with shape and electronic absorbance of Raman reporters along with laser sources. Hence, the effects of all these four parameters were systematically studied by changing either the different geometries/shapes of the gold substrates having a wide range of SPR or electronic absorbance of Raman reporters under a fixed laser excitation of 633 nm or 785 nm. Enhancement factor (EF) values were evaluated for each individual substrate with respect to five different Raman reporters which cover the maximum absorbance from 530 nm to 800 nm. The EF values suggest that the signal intensity has been increased by at least ten times compared to previous reports. Among the five different shapes of the gold nanoparticles, the substrate of gold nanostars (GNSt) was found to be the most suitable substrate for the highest signal enhancement under both excitation laser sources. The substrate of gold nanosphere (GNSp) is the second best at 633 nm laser excitation; however it is the modest substrate for SERS enhancement at 785 nm laser excitation. After finding the key tools for the development of ultrasensitive SERS nanoprobes, we selected the best Raman reporter (Cy7LA) for the development of biocompatible SERS nanotags in three different SERS substrates for the effective detection of cancer cells under 785 nm laser source.
Introduction
Surface-enhanced Raman spectroscopy (SERS) is a valuable analytical tool for the ultrasensitive identification and quantification of host of molecules ranging from chemical warfare agents, dyestuffs to biomolecules.1–3 Despite the substantial success of fluorescence based bio-imaging, the use of the technique is often limited by sensitivity, photostability and cytotoxicity.4–6 In the SERS technique, the use of the inelastic light scattering of noble metal nanoparticles paves the way for application of Raman spectroscopy due to its potentiality in overcoming the low sensitivity problem which is quite inherent in conventional Raman spectroscopy.7,8 Therefore, SERS techniques which provide a unique spectral fingerprint of a single molecule with high sensitivity have helped to overcome the two major concerns in the field of bio-imaging which are sensitivity and biocompatibility. Among the most common SERS substrates (Au, Ag, Cu), gold nanoparticles have been considered as the most suitable substrate for ultrasensitive bio-imaging platform due to their non-cytotoxicity, water solubility, long-term stability and good biocompatibility.9–12 Notable aspects of the SERS technique such as fruitfulness of spectroscopic information and high sensitivity towards bio-analytes, have motivated many studies which are associated with the design of gold substrates with different shape and size. Optically tuned SERS substrates involving nanorods, hollow nanostructures, nanostars and nanoflowers have been developed to create NIR-active hot spots to gain high sensitivity.13–18 Alternatively, 60 nm diameter nanospheres have been used to develop ultrasensitive NIR nanoprobes for the detection of tumor by applying NIR active Raman reporters which can resonance with NIR excitation laser source such as diode laser, 785 nm, to generate surface enhanced resonance Raman scattering (SERRS).19 Therefore, shape and surface plasmon resonance (SPR) of colloidal gold nanoparticles play a major role in the enhancement of the sensitivity of Raman scattering when it undergoes resonance with specific wavelength of Raman reporters under specific excitation laser source. A systematic study has been carried out on different sizes of the spherical nanoparticles, and it was found that 40–60 nm nanoparticles are preferable for obtaining the highest signal intensity.20–22 However, there has not yet been a systematic study to investigate the influence of the combination effects of both shapes and SPR of the gold substrates with absorbance wavelengths of Raman reporters and under a certain laser excitation source. Recently, Li et al. investigated the effect of the substrate shape (sphere, rod and star) on the surface-enhanced Raman scattering.23 However, their study was based on a 15 nm gold nanosphere substrate which is known to be a very poor gold substrate in view of the SERS intensity. Furthermore, their study was only focused on a single Raman reporter (MGITC) which was not suitable for SERRS study. Therefore, a systematic study on the four major parameters such as (a) shape of the substrates, (b) SPR of substrates, (c) electronic absorbance of Raman reporters and finally (d) excitation wavelength of the laser source may open a new window for the development of ultrasensitive nanoprobes. Herein, we report a systematic SERRS study using five gold substrates with different shapes along with variable LSPR and five different Raman reporters with variable absorption wavelengths to find out the most suitable combination for the development of ultrasensitive SERS nanotags. Furthermore, the most suitable Raman reporter has been applied to prepare ultrasensitive nanotags by varying three different substrate geometries such as gold nanosphere (GNSp), gold nanorod-800 (GNR-800), and gold nanostar (GNSt). These three different nanotags have been used in the detection of oral squamous cell carcinoma cells.
Result and discussion
Design
In this study, we have selected five cyanine dyes with different absorbance-wavelengths ranging from the visible to the near infrared (NIR) region. It is well known that cyanine dyes are good Raman active organic dyes.24–26 Our research group previously demonstrated that the incorporation of lipoic acid (LA) into the cyanine dyes was the key strategy for making suitable Raman reporters in the process of chemisorption on the surface of gold substrates.27 In this regard, we have chosen Cy3LA, Cy5LA, Cy7LA, Cy7.5LA and CyNAMLA-381 as Raman reporters.8,19,27,28 The structures of the five different Raman reporters are shown in Fig. 1a in which the alternative vinylene functional groups play a key role for the absorbance of light from the Vis-NIR regions. The maximum electronic absorbance of cyanine based Raman reporters are 540 nm, 645 nm, 750 nm, 740–790 nm, and 790 nm for Cy3LA, Cy5LA, Cy7LA, Cy7.5LA and CyNAMLA-381 respectively (Fig. 1b and S1†). These Raman reporters are considered to be an appropriate choice for the systematic study of wavelength dependent SERS signal's enhancement.
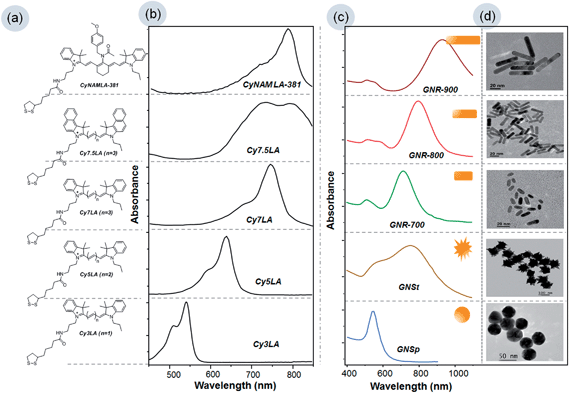 |
| Fig. 1 (a) & (b) Structure of Cy3LA, Cy5LA, Cy7LA, Cy7.5LA, CyNAMLA-381 and their corresponding Vis-NIR absorbance spectra in aqueous solution (540 nm, 645 nm, 750 nm, 740–790 nm and 790 nm). (c) SPR of GNSp, GNSt, GNR-700, GNR-800 and GNR900 and their corresponding (d) TEM images. | |
Furthermore, we also have chosen five different gold substrates: GNSp, GNR-700, GNR-800, GNR-900 and GNSt which display a wide range of surface plasmon resonance (SPR) to understand the effects of wavelength and geometry on the Raman enhancement. Fig. 1c displays the UV-Visible-NIR surface plasmon resonance curves of the gold substrates with different geometries (such as sphere, star and three different types of rods) and their corresponding TEM images are shown in Fig. 1d. According to the SPR, GNSp shows a maximum around 535 nm, and the three different gold GNRs show a maximum at 700 nm, 800 nm and 900 nm depending on the length of the nanorod, whereas GNSt covers the broad range from 500–900 nm. Eventually, the EF values of Raman reporters exhibit variable effects depending on the SPR value under identical condition of two different laser excitations (i.e. 633 nm and 785 nm).
Synthesis
Herein, we used 60 nm GNSp, and three different GNRs such as GNR-700, GNR-800 and GNR-900 and GNSt were prepared according to the well-documented seed-mediated growth methods.29–35 Five different Raman reporters were synthesized according to our previously reported methods.19,27,28
Enhancement factor (EF)
Applying different combinations of gold substrates and Raman reporters, we measured the EF values under visible and NIR laser excitation wavelengths. Here, we fixed the concentrations of Raman reporters and then varied the concentrations of the gold substrates in order to reach the saturation point. As different shapes of gold substrates are stabilized with different surfactants, sodium citrate stabilized GNSp, and polyvinylpyrrolidone (PVP) coated GNSt were incubated for 10 min for the replacement by lipoic acid of Raman reporters. However, CTAB stabilized GNRs was incubated overnight. The spectra were recorded after complete adsorption of the dyes on the nanoparticles. The maximum SERS enhancement was found at concentration of all the gold substrates as follows: 6.5 nM for GNSp, 1.8 nM for GNRs and 6 nM for GNSt (Fig. S3†). At this saturation point, we calculated the enhancement factor (EF) of five different Raman reporters with each gold substrate. Thus, the calculated EF values provide a clear picture to investigate the correlation between the different parameters and the signal enhancement effect. The EF was calculated according to the following eqn (1) (ref. 23, 36 and 37) in which the maximum peak intensity of each signature molecule was considered at 552, 555, 503, 586, and 523 cm−1 for Cy3LA, Cy5LA, Cy7LA, Cy7.5L and CyNAMLA-381 respectively (Fig. S2†). |
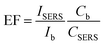 | (1) |
In eqn (1), ISERS and CSERS are the Raman intensities and the molar concentrations of our signature molecules (Cy3LA, Cy5LA, Cy7LA, Cy7.5LA and CyNAMLA-381) in the presence of gold nanoparticles. Similarly, Ib and Cb are the Raman intensities and the molar concentrations of the signature molecules in the absence of gold nanoparticles.
Table 1 and Fig. 2 clearly indicate that the EF values are in order of 105 orders at 785 nm laser excitation and 104 orders at 633 nm excitation. We have seen that the trend of the relative Raman signal enhancement among different shapes is similar to what has been reported previously i.e. nanostars > nanorods > nanospheres. However, the EF values are ten times higher than those of earlier reports. These high EF values were achieved in these experiments due to the combination effects of resonance with excitation laser source and the electronic absorbance of Raman reporters along with SPR of gold substrates. Furthermore, different shapes contribute their inherent alignment to generate maximum electric field intensity and generation of “hot spots”. Henceforth, we discussed the effects of each parameter in more details.
Table 1 EF value calculation of five different Raman reporters with respect to five different gold substrates under 785 nm and 633 nm laser excitation wavelengths
Dye |
|
Cy-3 LA |
Cy-5 LA |
Cy-7LA |
Cy-7.5LA |
CyNAMLA 381 |
Au nanostructure (surface plasmon) |
|
633 nm (×104) |
785 nm (×105) |
633 nm (×104) |
785 nm (×105) |
633 nm (×104) |
785 nm (×105) |
633 nm (×104) |
785 nm (×105) |
633 nm (×104) |
785 nm (×105) |
Sphere (540 nm) |
0.91 |
0.15 |
0.88 |
0.18 |
0.46 |
2.6 |
0.41 |
1.5 |
0.08 |
2.1 |
Nanorod (700 nm) |
0.06 |
0.19 |
0.008 |
0.23 |
0.05 |
2.8 |
0.02 |
1.9 |
0.015 |
1.8 |
Nanorod (800 nm) |
0.02 |
0.23 |
0.005 |
0.20 |
0.006 |
5.2 |
0.01 |
3.2 |
0.02 |
3.1 |
Nanorod (900 nm) |
0.03 |
0.21 |
0.002 |
0.18 |
0.005 |
4.1 |
0.015 |
4.2 |
0.003 |
4.2 |
Nanostar (600–900 nm) |
0.59 |
0.33 |
1.21 |
0.23 |
2.46 |
6.9 |
1.06 |
5.8 |
2.71 |
5.7 |
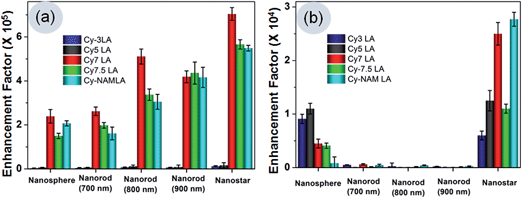 |
| Fig. 2 (a) Relative SERS intensity among the different geometry of the gold substrates under the excitation laser source at 785 nm (b) under the excitation laser source at 633 nm. Five different Raman reporters contribute to the enhancement of Raman intensity by resonance of their electronic absorbance with substrates SPR and laser. | |
Effects of absorbance of Raman reporters on EF
Table 1 results suggest that the electronic absorbance of reporters play important roles for signal enhancement. Visible light absorbing Cy3LA and Cy5LA showed the least enhancement compare to NIR light absorbing Cy7LA, Cy7.5LA and CyNAMLA-381 (Fig. 2), under the 785 laser source. This is due to the resonance between electronic absorbance of NIR Raman reporters and NIR laser source. Interestingly, we found that Cy7LA showed the highest EF compared to other NIR light absorbing reporter molecules. Here, the possible reason could be attributed to the minimum energy dissipation via fluorescence incidence. However, the other two NIR signature molecules (Cy7.5LA and CyNAMLA-381) lose partial energy through its emission under excitation laser source at 785 nm.
Effects on EF of surface plasmon resonance of gold substrates
SPR of gold substrates show also an effective role for signal enhancement along with the absorbance of reporters. Among the three different types of gold nanorods, the enhancement effect in GNR-700 was much weaker than those of its other counterparts (GNR-800 and GNR-900) due to the lower resonance efficiency of the 785 nm laser. Here, GNR-800 is expected to show a better signal due to its perfect overlap with 785 nm excitation laser source however, we observed that GNR-900 is a little better than GNR-800. This can be explained in a way that GNR-900 was dominating its inherent alignment to generate maximum electric field intensity rather than the resonance with the excitation laser source. The additional weaker SPR bands of GNRs, which appeared around 530 nm wavelength, can partially contribute to resonance with the 633 nm excitation laser. Among the three types of GNRs, GNR-700 is having partial SPR appearance at 633 nm whereas the other two GNRs do not have any SPR at the same wavelengths. As a consequence, the SERS intensity due to the resonance effect of GNR-700 at 633 laser excitation is little better than the other GNRs. Furthermore, GNSt showed the highest EF values compare to GNRs or GNSp with both excitation laser sources at 785 nm or 633 nm. This phenomenon is due to the broad localized SPR of the nanostars ranging from 500 nm to 900 nm along with the characteristic shape.
Effects of the shape of the gold substrates
The special shape of the nanostars plays the most vital role in generating the maximum electric field intensity, which causes the highest signal intensity with both laser excitations. The maximum electromagnetic field intensity which was intensified at the sharp tips of the nanostars developed SPR at NIR regions around 700–800 nm.23,38–40 This defined localized SPR of GNSt can generate “hot spots” around the sharp tips, resulting in highly sensitive SERS signals under 785 nm laser source. Furthermore, another shoulder band which is due to the surface plasmon resonance (SPR) of the sphere like central core structure of GNSt contributes to enhance Raman scattering at 633 nm laser excitation. For gold nanorods, maximum electromagnetic field which was concentrated at its two longitudinal ends, qualify the highest SERS intensity after the resonance with 785 nm laser.41–43 Interestingly, the result indicates that the longitudinal ends of the nanorods align parallel to the NIR laser polarization, causing the most significant SERS enhancement. However, GNSp lacks the advantages which are present in GNRs or GNSt. Therefore, it has the least structural effects to induce the SERS enhancement in identical experimental conditions. After considering the effects of different parameters on SERS, we conclude that the most sensitive NIR Raman reporter is Cy7LA among others and nanostar was the most suitable shape of substrate for the generation of NIR “hot spots” to develop NIR ultrasensitive nanoprobes. Therefore, this Cy7LA was further applied for cancer cells imaging by changing the different substrates from sphere, star to rods.
Cytotoxicity. Gold nanoparticles are known to be the less toxic metal nanoparticles compared to silver and other heavy metals ones. Therefore, extensive research studies have been focused on the development of nanoprobes based on gold nanoparticles. We have previously demonstrated the suitability of nanoprobes for in vivo imaging and carried out a kinetics study for 8 days to demonstrate the biocompatibility.28 In this study, we performed a cytotoxicity assay in OSCC cell line to validate the biocompatibility of these SERS nanotags for in vitro systems. We have considered few representative combinations to test the cell viability by applying the CCK-8 assay. We represent here two dyes (Cy-7LA and Cy7.5LA) with various concentrations (4 and 2 μM) and three different set of nanotags such as GNSp@Cy-7LA@PEG, GNSt@Cy-7LA@PEG, GNR@Cy-7LA@PAA and GNSp@Cy-7.5LA@PEG. Interestingly, we found that the Au-conjugates and even the dye alone did not show high cytotoxicity; the cells are almost >90% viable with respect to control (Fig. 3)
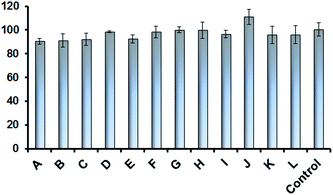 |
| Fig. 3 Cell viability of OSCC cells after 3 h incubation with (A) GNSp@Cy-7.5LA@PEG (5.66 × 10−8 M); (B) GNSp@Cy-7.5LA@PEG (2.88 × 10−8 M); (C) Cy-7LA (4 × 10−6 M); (D) Cy-7LA (2 × 10−6 M); (E) GNSp@Cy7LA@PEG (5.66 × 10−8 M); (F) GNSp@Cy-7LA@PEG (2.88 × 10−8 M); (G) GNSt@Cy7LA@PEG (2.5 × 10−8 M); (H) GNSt@Cy-7LA@PEG (1.25 × 10−8 M); (I) Cy-7.5LA (4 × 10−6 M); (J) Cy-7.5LA (2 × 10−6 M); (K) GNR@Cy-7LA@PAA (2.5 × 10−8 M); (L) GNR@Cy-7LA@PAA (1.25 × 10−8 M); control: PBS buffer without any dye or gold nanoparticles. | |
Cancer cells imaging
Now, using the most suitable Raman reporter (Cy7LA), we prepared three different nanotags named as Cy7LA@GNSp@PEG-anti-EGFR, Cy7LA@GNR-800@PAA-anti-EGFR and Cy7LA@GNSt@PEG-anti-EGFR for inspecting target specific recognition in live cancer cells. For the preparation of the biocompatible SERS nanotags, the gold substrates were saturated with the Cy7LA reporter, and then coated with mixed PEG or poly acrylic acid (PAA) ligands. It should be noted that PAA, an encapsulating agent, was applied to the nanorods instead of PEG as the latter was not effective to replace the CTAB even after 24 h incubation. After encapsulation of PEG or PAA in the nanoparticles, these were characterized by TEM images. There was no aggregation among the particles (Fig. S5†). Next, these stable biocompatible nanotags were conjugated with specific targeted antibody i.e. anti-EGFR for targeting epidermal growth factor receptors (EGFR), over expressed receptors on the cell membrane in oral squamous cell carcinoma (OSCC) cells.4,10,44–46 After successful conjugation of antibody through amide coupling, these nanotags were characterized by means of measurement of the SERS intensity as well as absorbance spectra of proteins at 280 nm (Fig. S4†). Finally, we incubated the antibody modified SERS nanotags to the cells and scanned at 503 cm−1 and 554 cm−1 to get clear images of specific cancer cells from the cell surface receptors (Fig. 4). Contrary, anti-HER2 conjugated nanoparticles does not show any significant SERS signal from cell surfaces (Fig. S6†).
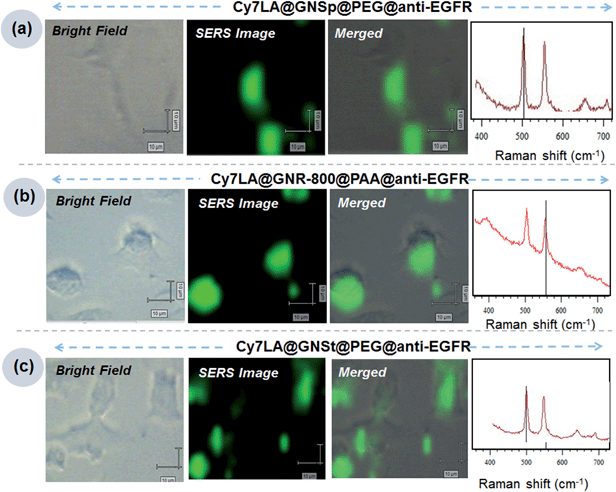 |
| Fig. 4 (a) OSCC cell images by SERS nanotags (Cy7LA@GNSp@PEG-anti-EGFR): in a row BF image of OSCC cells; SERS image at 503 cm−1 and merged images. (b) OSCC cell images by SERS nanotags (Cy7LA@GNR-800@PAA-anti-EGFR): in a row BF image of OSCC cells; SERS image at 554 cm−1 and merged images. (c) OSCC cell images by SERS nanotags (Cy7LA@GNSt@PEG-anti-EGFR): in a row BF image of OSCC cells; SERS image at 503 cm−1 and merged images. | |
We successfully demonstrated the specific recognition of OSCC cells by using three individual nanotags. The cell mapping experiment clearly confirms that these nanotags are capable of specific recognition of cell surface receptors on the cell membranes. Moreover, these cell mapping images correlated well with the previously reported results.47 These experiments were performed by using a minimum amount of nanotags (final concentration of nanotags: 10 μL × 400 pM diluted to 500 μL final volume: i.e. 8 pM). The concentrations used here was much lower compared to previous reports.8 For the proof of concept, we have presented here a cancer cell imaging application using these new biocompatible SERS probes.
Conclusions
We reported a systematic study on SERS signal enhancement resulting from the variance of four parameters that play a major role for enhancing SERS signals. Here, we successfully demonstrated that the selection of the appropriate combination of four major parameters such as the geometry of the gold substrates, LSPR of the gold substrates, electronic absorption wavelengths of the Raman reporters and laser sources, is the key for the development of ultrasensitive SERS nanotags. From this study, it is obvious that the SERS signal enhancement was strongly dependent on the wavelength of the Raman reporters and LSPR of the gold substrates along with the geometry of the substrates. The most suitable combination for the development of an ultrasensitive NIR SERS probe is substrate of gold nanostars along with Cy7LA as Raman reporter. The second and third most suitable conditions are GNR-900 and GNR-800 along with Cy7LA. The least suitable NIR SERS nanoprobes can be prepared by the combination of 60 nm GNSp along with Cy7LA. Nanostar substrates can be used as the most suitable candidate for visible SERS probe development. Nanospheres are much better than nanorods when choosing Raman reporters as visible light absorbing candidates. The new findings presented in this systematic study may help developing ultrasensitive SERS nanoprobes in the future. For the proof of concept, we have shown here a cancer cell imaging application with these new biocompatible SERS probes.
Acknowledgements
We gratefully acknowledge the A*STAR Joint Council Office (JCO), Singapore (Grant 1231AFG028) for financial support.
References
- M. Leona, J. Stenger and E. Ferloni, J. Raman Spectrosc., 2006, 37, 981–992 CrossRef CAS
. -
(a) Y. S. Huh, A. J. Chung, B. Cordovez and D. Erickson, Lab Chip, 2009, 9, 433–439 RSC
;
(b) K. Kneipp, H. Kneipp, I. Itzkan, R. R. Dasari and M. S. Feld, J. Phys.: Condens. Mater., 2002, 14, R597–R624 CrossRef CAS
. - C. David, N. Guillot, H. Shen, T. Toury and M. L. de la Chapelle, Nanotechnology, 2010, 21, 475501 CrossRef PubMed
. - X. M. Qian, X. H. Peng, D. O. Ansari, Q. Yin-Goen, G. Z. Chen, D. M. Shin, L. Yang, A. N. Young, M. D. Wang and S. M. Nie, Nat. Biotechnol., 2008, 26, 83–90 CrossRef CAS PubMed
. - S. Kim, Y. T. Lim, E. G. Soltesz, A. M. De Grand, J. Lee, A. Nakayama, J. A. Parker, T. Mihaljevic, R. G. Laurence, D. M. Dor, L. H. Cohn, M. G. Bawendi and J. V. Frangioni, Nat. Biotechnol., 2004, 22, 93–97 CrossRef CAS PubMed
. - M. N. Rhyner, A. M. Smith, X. Gao, H. Mao, L. Yang and S. Nie, Nanomedicine, 2006, 1, 209–217 CrossRef CAS PubMed
. - W. J. Cho, Y. Kim and J. K. Kim, ACS Nano, 2012, 6, 249–255 CrossRef CAS PubMed
. - K. K. Maiti, A. Samanta, M. Vendrell, K. S. Soh, M. Olivo and Y. T. Chang, Chem. Commun., 2011, 47, 3514–3516 RSC
. - K. K. Maiti, U. S. Dinish, C. Y. Fu, J. J. Lee, K. S. Soh, S. W. Yun, R. Bhuvaneswari, M. Olivo and Y. T. Chang, Biosens. Bioelectron., 2010, 26, 398–403 CrossRef CAS PubMed
. - S. Lee, H. Chon, S. Y. Yoon, E. K. Lee, S. I. Chang, D. W. Lim and J. Choo, Nanoscale, 2012, 4, 124–129 RSC
. - B. Sharma, R. R. Frontiera, A. I. Henry, E. Ringe and R. P. Van Duyne, Mater. Today, 2012, 15, 16–25 CrossRef CAS
. - T. Vo-Dinh, F. Yan and M. B. Wabuyele, J. Raman Spectrosc., 2005, 36, 640–647 CrossRef CAS
. - K. D. Alexander, K. Skinner, S. P. Zhang, H. Wei and R. Lopez, Nano Lett., 2010, 10, 4488–4493 CrossRef CAS PubMed
. - B. Nikoobakht and M. A. El-Sayed, J. Phys. Chem. A, 2003, 107, 3372–3378 CrossRef CAS
. - C. Hrelescu, T. K. Sau, A. L. Rogach, F. Jäckel, G. Laurent, L. Douillard and F. Charra, Nano Lett., 2011, 9, 402–407 CrossRef PubMed
. - D. Xu, J. J. Gu, W. N. Wang, X. C. Yu, K. Xi and X. D. Jia, Nanotechnology, 2010, 21, 375101 CrossRef PubMed
. - Q. Li, Y. Y. Jiang, R. C. Han, X. L. Zhong, S. Y. Liu, Z. Y. Li, Y. L. Sha and D. S. Xu, Small, 2013, 9, 927–932 CrossRef CAS PubMed
. - C. G. Khoury and T. Vo-Dinh, J. Phys.
Chem. C, 2008, 112, 18849–18859 CAS
. - A. Samanta, K. K. Maiti, K. S. Soh, X. J. Liao, M. Vendrell, U. S. Dinish, S. W. Yun, R. Bhuvaneswari, H. Kim, S. Rautela, J. H. Chung, M. Olivo and Y. T. Chang, Angew. Chem., Int. Ed., 2011, 50, 6089–6092 CrossRef CAS PubMed
. - N. Nath and A. Chilkoti, Anal. Chem., 2004, 76, 5370–5378 CrossRef CAS PubMed
. - V. Joseph, A. Matschulat, J. Polte, S. Rolf, F. Emmerling and J. Kneipp, J. Raman Spectrosc., 2011, 42, 1736–1742 CrossRef CAS
. - S. Link and M. A. El-Sayed, J. Phys. Chem. B, 1999, 103, 4212–4217 CrossRef CAS
. - M. Li, S. K. Cushing, J. Zhang, J. Lankford, Z. P. Aguilar, D. Ma and N. Wu, Nanotechnology, 2012, 23, 115501 CrossRef PubMed
. - W. Leng and A. M. Kelley, J. Am. Chem. Soc., 2006, 128, 3492–3493 CrossRef CAS PubMed
. - K. K. Maiti, A. Samanta, M. Vendrell, K. S. Soh, M. Olivo and Y. T. Chang, Chem. Commun., 2011, 47, 3514–3516 RSC
. - G. von Maltzahn, A. Centrone, J. H. Park, R. Ramanathan, M. J. Sailor, T. A. Hatton and S. N. Bhatia, Adv. Mater., 2009, 21, 3175–3180 CrossRef CAS PubMed
. - K. K. Maiti, U. S. Dinish, C. Y. Fu, J. J. Lee, K. S. Soh, S. W. Yun, R. Bhuvaneswari, M. Olivo and Y. T. Chang, Biosens. Bioelectron., 2010, 26, 398–403 CrossRef CAS PubMed
. - K. K. Maiti, U. S. Dinish, A. Samanta, M. Vendrell, K. S. Soh, S. J. Park, M. Olivo and Y. T. Chang, Nano Today, 2012, 7, 85–93 CrossRef CAS PubMed
. - X. C. Ye, L. H. Jin, H. Caglayan, J. Chen, G. Z. Xing, C. Zheng, D. N. Vicky, Y. J. Kang, N. Engheta, C. R. Kagan and C. B. Murray, ACS Nano, 2012, 6, 2804–2817 CrossRef CAS PubMed
. - P. S. Kumar, I. Pastoriza-Santos, B. Rodriguez-Gonzalez, F. J. Garcia de Abajo and L. M. Liz-Marzan, Nanotechnology, 2008, 19, 15606 CrossRef PubMed
. - M. Schutz, D. Steinigeweg, M. Salehi, K. Kompe and S. Schlucker, Chem. Commun., 2011, 47, 4216–4218 RSC
. - M. Yamamoto, Y. Kashiwagi, T. Sakata, H. Mori and M. Nakamoto, Chem. Mater., 2005, 17, 5391–5393 CrossRef CAS
. - S. E. Lohse and C. J. Murphy, Chem. Mater., 2013, 25, 1250–1261 CrossRef CAS
. - A. M. Gabudean, D. Biro and S. Astilean, Nanotechnology, 2012, 23, 485706 CrossRef CAS PubMed
. - N. R. Jana, L. Gearheart and C. J. Murphy, J. Phys. Chem. B, 2001, 105, 4065–4067 CrossRef CAS
. - E. C. Le Ru, E. Blackie, M. Meyer and P. G. Etchegoin, J. Phys. Chem. C, 2007, 111, 13794–13803 CAS
. - P. H. C. Camargo, C. M. Cobley, M. Rycenga and Y. N. Xia, Nanotechnology, 2009, 20, 434020 CrossRef PubMed
. - C. G. Khoury and T. Vo-Dinh, J. Phys. Chem. C, 2008, 112, 18849–18859 CAS
. - S. K. Dondapati, T. K. Sau, C. Hrelescu, T. A. Klar, F. D. Stefani and J. Feldmann, ACS Nano, 2010, 4, 6318–6322 CrossRef CAS PubMed
. - J. Henzie, J. E. Barton, C. L. Stender and T. W. Odom, Acc. Chem. Res., 2006, 39, 249–257 CrossRef CAS PubMed
. - J. Aizpurua, G. W. Bryant, L. J. Richter, F. J. G. de Abajo, B. K. Kelley and T. Mallouk, Phys. Rev. B: Condens. Matter Mater. Phys., 2005, 71, 235420 CrossRef
. - A. M. Funston, C. Novo, T. J. Davis and P. Mulvaney, Nano Lett., 2009, 9, 1651–1658 CrossRef CAS PubMed
. - S. L. Smitha, K. G. Gopchandran, T. R. Ravindran and V. S. Prasad, Nanotechnology, 2011, 22, 265705 CrossRef CAS PubMed
. - K. Yang, F. J. Zhang, H. Tang, C. Zhao, Y. A. Cao, X. Q. Lv, D. Chen and Y. D. Li, Int. J. Nanomed., 2011, 6, 1739–1745 CrossRef CAS PubMed
. - S. A. Sarkis, B. H. Abdullah, B. A. A. Majeed and N. G. Talabani, Head Neck Oncol., 2010, 2, 13 CrossRef PubMed
. - V. F. Bernardes, F. O. Gleber-Netto, S. F. Sousa, T. A. Silva and M. C. F. Aguiar, J. Exp. Clin. Cancer Res., 2010, 29, 40 CrossRef PubMed
.
Footnotes |
† Electronic supplementary information (ESI) available. See DOI: 10.1039/c3ra46208k |
‡ A. Samanta and S. Jana have contributed equally to this work. |
|
This journal is © The Royal Society of Chemistry 2014 |
Click here to see how this site uses Cookies. View our privacy policy here.