DOI:
10.1039/C3RA47794K
(Paper)
RSC Adv., 2014,
4, 17709-17716
DFT study on effect of CO on the system of acetoxylation of ethylene to vinyl acetate
Received
4th January 2014
, Accepted 21st March 2014
First published on 28th March 2014
Abstract
We report the competitive adsorption of CO, ethylene and acetate on a Pd–Au (100) surface in the reaction system of vinyl acetate synthesis with DFT method. The effect of CO on the catalytic performance of Pd–Au in the vinyl acetate system was also studied. Moreover, the method of reducing the content of CO in the industrial process and the reaction path of CO on the Pd–Au catalyst was investigated. Furthermore, the effect of CO on the acetoxylation of ethylene to vinyl acetate was reflected in two aspects. Firstly, the adsorption of CO on a Pd atom is more stable than that of ethylene, in which the adsorption of ethylene is blocked and the catalytic activity of the Pd–Au alloy decreases. Secondly, the Pd–Au alloy can catalyze the CO oxidation reaction.
1. Introduction
Vinyl acetate (VA) plays an important role in organic raw materials and is usually utilized as a chemical intermediate in surface coatings, adhesives and paints. It has an important position in the development of the national economy. With the global development of the petrochemical industry, the acetoxylation of ethylene has gradually replaced the acetylene method and become the dominant commercial route for the synthesis of VA because of its technological and economical advantages.1–4 Especially, the technological breakthrough on the industrial-scale ethylene formation from bio-ethanol5,6 has made bio-ethylene more attractive to VA producers compared to the traditional fossil fuel ethylene source. However, the mechanism of the catalyst is still ambiguous, especially with respect to the existence of CO.
Pd–Au alloys have attracted increased attention because of their markedly different properties toward various reactions such as the synthesis of VA.7–11 Chen and his group12 summarized the understanding of the effect of Au in a Pd–Au catalyst for VA synthesis. They believed that Au atoms broke the contiguous Pd atom ensembles at the surface into isolated Pd atoms, and then these Pd sites significantly reduced the formation of by-products and suppressed CO poisoning. As we all know, surface composition plays an important role for understanding the role of alloying in bimetallic catalysts. Based on the investigation of Chen,13 when the coverage rate of Pd atoms in Pd–Au(100) is 0.1, VA conversion achieves better results than in the case of Pd–Au(111). Thus, in this study, a model surface of Pd–Au(100) was chosen to investigate the active sites of a Pd–Au mixture and the surface reaction.
It is well known that CO as a micro-molecule can easily adsorb on the surfaces of the metal catalysts. Calaza14 found out that the CO oxidation reaction occurred only on a surface with contiguous Pd sites, monitored using both reaction kinetic measurements and PM-IRAS. Wei15 studied the chemisorptive behavior of CO on a Pd–Au mixture by infrared reflection-adsorption spectroscopy (IRAC). Considering that there is CO with ethylene in the upper stream products, which affects the activity of the catalyst, it is important to find out the effect of CO on a VA system at the electron level. Thus, in this paper, the density functional theory (DFT) method was carried out to investigate the thermal stability of the Pd–Au alloy, the mono-adsorption properties of ethylene and acetate, and the co-adsorption of the reactants with CO, which helps to investigate the effect of CO on the acetoxylation of ethylene to VA.
2. Computational methodology and models
2.1 Computational methodology
Using the generalized gradient approximation (GGA) with RPBE16 as the exchange-correlation functional, we carried out the calculations with the program package DMol3 in Material Studio of Accelrys. To maintain the balance between computational accuracy and efficiency, the core treatment was set with DFT semi-core pseudo-potential (DSPP). The electron density was converged to within 1.0 × 10−5 eV, and the geometry optimizations were performed with DFT until the force on each atom was less than 0.004 Ha Å−1 by using smearing at 0.005 Ha. The adsorption energy (Ead(M)) and co-adsorption energy (Ead(B)) of different reactant species on different Pd–Au(100) alloy surfaces were individually defined as follows:
Ead(M) = EPdAu + EM − EM/PdAu, |
Ead(B) = EA/PdAu + EB − E(A+B)/PdAu, |
where Ead(M) is the adsorption energy of every species and Ead(B) is the total energy of different reactant species adsorbed on the corresponding Pd–Au surface slabs, the bare Pd–Au surface slabs and the free reactant species.
2.2 Models
Firstly, ethylene and acetic acid molecules were built, and then stable structures were obtained through structural optimization. Taking the optimized molecules into the model of the Pd–Au(100) surface, we carried out structural optimization to obtain a stable adsorption system of ethylene and acetic acid. Based on the adsorption structure of acetate with structural convergence on the Pd–Au(100) surface, the hydrogen atom from the hydroxyl oxygen of the acid molecule was further taken off. Then, the corresponding adsorption system of acetate and the co-adsorption system of ethylene and acetate were obtained by structural optimization.
3. Results and discussion
3.1 Ethylene
3.1.1 Adsorption geometry of ethylene. The Pd–Au(100) surface was built using a 4 × 4 unit cell composed of four layers of atoms with 15 Å of vacuum in the z direction. On these surfaces, different sets of Au atoms were replaced by Pd atoms similar to the configurations shown in Fig. 1. The k-point sampling consisted of 2 × 2 × 1 Monkhorst–Pack points.17–20 Considering that the computational results agreed well with the experimental data and time consumption, the precision with the 2 × 2 × 1 k-points was acceptable compared to that with the other k-points.
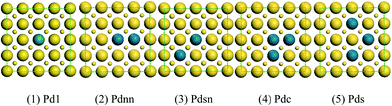 |
| Fig. 1 Different ensembles and adsorption sites on the Pd–Au(100) surface. Yellow balls represent Au, and blue ones are Pd atoms such as Pd1 and Pdnn. Each image represents a different geometry. The different sizes of the yellow balls represent the different layers. | |
The two surface upper layers were optimized while the lowest layers were kept frozen at the bulk distances.
Based on the stable Pd–Au geometry, we investigated the most stable adsorption ensembles of ethylene on the Pd–Au surfaces. While experimental21 and theoretical22 investigations have shown that ethylene can adsorb on Pd sites with both di-σ-bonded and π-bonded configurations, the details of geometric and electronic effects on them are still unclear. In accordance with the previous evidence, as shown in Fig. 2 (only examples of Pdnn), the DFT calculations performed in this paper have demonstrated that only the π-bonded configuration can steadily adsorb on discontinuous Pd atoms while both di-σ-bonded and π-bonded ethylene species can steadily adsorb on Pd sites with continuous Pd atoms.
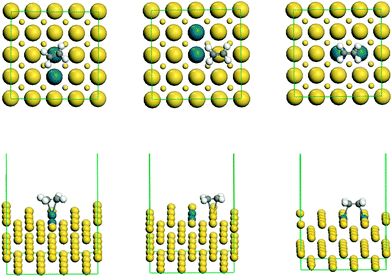 |
| Fig. 2 The stable adsorption geometry of ethylene in the Pdnn ensemble. | |
3.1.2 The PDOS of the π-bond and di-σ-bond ethylene species. Although the stable adsorption geometries of ethylene have been obtained, the interaction between ethylene species and metal atoms is unknown. Therefore, by studying the PDOS of the π-bond and di-σ-bond ethylene species on the Pdnn surface, the interaction between ethylene and the metal surface is illustrated as shown in Fig. 3.
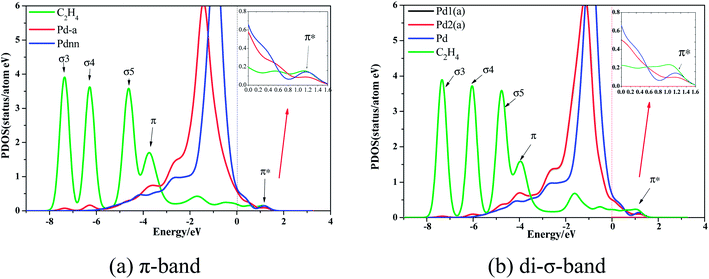 |
| Fig. 3 PDOS of ethylene and Pdnn ensembles on Pd–Au(100) surface. | |
Compared with the d-band PDOS curve of Pd without ethylene adsorption, the d-band PDOS curve with ethylene adsorption obviously moves toward the left direction. In addition, Fig. 3 shows that the overlapping peaks of the σ3, σ4, and σ5 orbitals and Pd d-band PDOS are small, but they are larger in the π-orbital. Moreover, the PDOS curve of Pd-adsorbed ethylene moves lower compared to Pd without ethylene adsorption in the π*-orbital. These changes illustrate that the relationship between ethylene and the Pd atom of the Pd surface is mainly the interactions between the π- and π*-orbital and the Pd atom's d-band, when ethylene adsorbs on the Pd surface.
The contrast between Fig. 3(a) and (b) shows that ethylene's PDOS curve of different adsorption configurations changes little, which demonstrates that the interaction mechanism between different ethylene adsorption configurations and Pd atoms is consistent.
3.2 CO and ethylene
The stable structure of co-adsorption between CO and ethylene on the surface of Pdnn, Pdsn and Pdc ensembles is shown in Fig. 4.
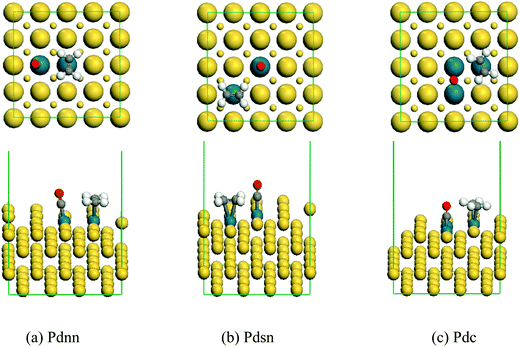 |
| Fig. 4 The stable co-adsorption geometry of ethylene and CO on the surface of Pdnn, Pdsn and Pdc ensembles. | |
The stable co-adsorption energy of ethylene and CO on the surface of the Pdnn, Pdsn and Pdc ensembles is shown in Fig. 5.
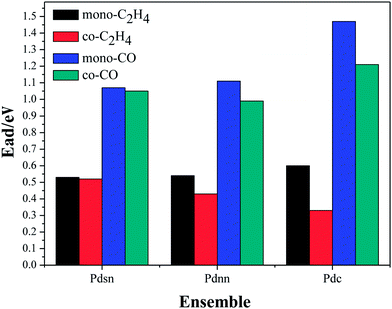 |
| Fig. 5 The co-adsorption energy of ethylene and CO on the surface of the Pdnn, Pdsn and Pdc ensembles. | |
From Fig. 4, we can know that ethylene and CO adsorb on the Pd active sites, separately, which is in accordance with single molecule adsorption. As shown in Fig. 5, the adsorption energy of CO is larger than that of ethylene in the system of vinyl acetate production. Therefore, it will impact the ethylene adsorption, and then suppress the reaction of acetate with ethylene when CO occupies the active site. Thus, ethylene can adsorb on the active site and react with acetic acid in the case of trace CO amounts. However, CO may occupy more active sites than ethylene with an increasing amount of CO in the reaction system, which influences the catalyst activity and even results in catalyst deactivation. In addition, the adsorption energy of both ethylene and CO decreases in different degrees compared with the single molecule adsorption energy. This tells us the molecular repulsive force has a little impact on the adsorption energy.
3.3 Acetate
3.3.1 The stable adsorption geometry of acetate on Pdnn ensemble. As one of the critical reactants in the synthesis of vinyl acetate, acetic acid can easily dissociate into acetate, which can adsorb stably on the surface in the presence of O2.The stable structure of acetate on the surface is also studied as shown in Fig. 6. In the non-continuous Pd surface configuration (a), there is only one stable form of the C–C bond with perpendicular adsorption, whereas in a continuous Pd structure surface (b), there are two stable forms of the C–C bond with vertical adsorption.
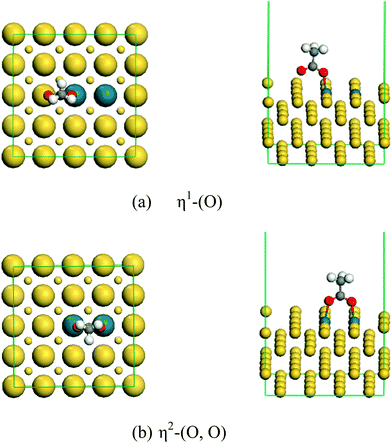 |
| Fig. 6 The stable adsorption geometry of acetate on the Pdnn ensemble. | |
η1-(O) represents an O atom adsorbed on a Pd atom; η2-(O, O) represents two O atoms are adsorbed on a Pd atom.
3.3.2 The PDOS of acetate and the Pdnn ensemble. The acetate adsorbed on the catalyst surface will form a chemical bond between catalysts, which plays a role in the electron transfer. As shown in Fig. 7, we found that there was a larger change in the p orbital PDOS curve of O atoms—before and after acetate adsorption—than in the other orbits; thus, the following figure shows the PDOS curve of the p orbital (two O atoms of acetate) and the d-band of Pd or Au, which is helpful to understand the electronic interaction between acetate and the surface.
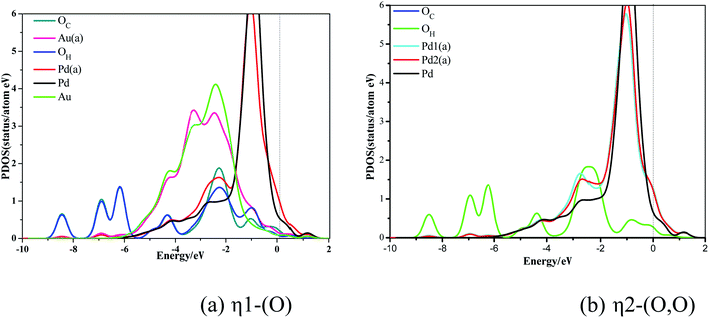 |
| Fig. 7 The PDOS of acetate and the Pdnn ensemble. | |
3.4 CO and acetate
The stable co-adsorption geometries of acetate and CO on the surface of the Pdnn, Pdsn and Pdc ensembles are shown in Fig. 8.
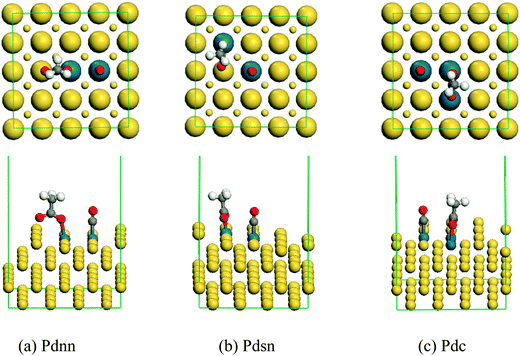 |
| Fig. 8 The stable co-adsorption geometry of acetate and CO on the surface of the Pdnn, Pdsn and Pdc ensembles. | |
The stable co-adsorption energy of acetate and CO on the surface of the Pdnn, Pdsn and Pdc ensembles is shown in Fig. 9.
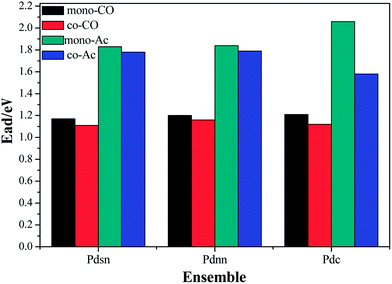 |
| Fig. 9 The co-adsorption energy and mono-adsorption energy of acetate and CO on the surface. | |
In the stable adsorption configuration shown in Fig. 8, acetate and CO occupy the active centers. According to the adsorption energy data shown in Fig. 9, the adsorption energy of acetate with the same configuration surface is larger than that of CO by about 0.7 eV. Therefore, the adsorption configuration of acetate is more stable than that of CO, and the adsorption intensity is higher. Combined with the co-adsorption energy of ethylene and CO shown in Fig. 5, the co-adsorption energy in different configuration surfaces decreases slightly. In the process of vinyl acetate formation from ethylene, the rate-controlling step is the coupling process of ethylene to vinyl acetate–Pd intermediate with acetate. However, as CO possesses more adsorption capacity and its molecular size is smaller than that of ethylene, it is easier to occupy Pd active sites, which has a certain impact on the synthesis of vinyl acetate. CO may occupy more active sites than ethylene with the amount of CO increasing in the reaction system, which influences the rate-controlling step of the reaction and then reduces the catalyst activity and even results in catalyst deactivation.
As shown in Fig. 9, the co-adsorption energy of the acetate and CO is less in all the configurations than in the monomolecular adsorption; however, the degree of reduction in surface Pdsn is less than that in the surface Pdnn and Pdc, which is due to the distance between the Pd atoms on the surface. Further, the closer distance between the Pd atoms leads to a smaller repulsive force of co-adsorption between molecules; therefore, it has little impact on the adsorption energy.
3.5 CO–ethylene–acetate
The active site of Pd–Au is Pd, so we only considered the co-adsorption of acetate, ethylene and CO in the surface configurations of three Pd atoms including Pds and Pdc. The stable co-adsorption geometry of acetate, ethylene and CO on the surface of the Pds and Pdc ensembles is shown in Fig. 10.
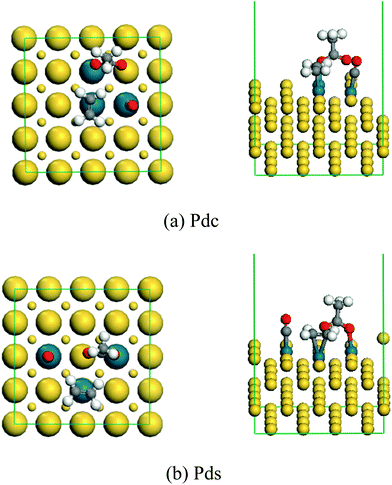 |
| Fig. 10 The stable co-adsorption geometry of acetate, ethylene and CO on the surface of the Pds and Pdc ensembles. | |
The stable co-adsorption energy of acetate, ethylene and CO on the surface of the Pds and Pdc ensembles is shown in Fig. 11.
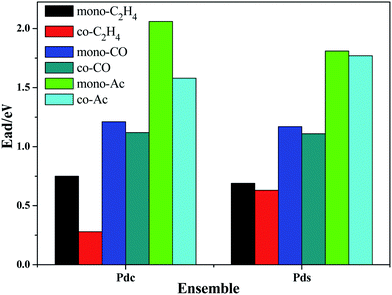 |
| Fig. 11 The co-adsorption energy of CO, ethylene and acetate on the surface of the Pdsn and Pdc ensembles. | |
The co-adsorption data of CO, ethylene and acetate confirms that CO would affect the reaction system of vinyl acetate, once again. After the co-adsorption of acetate and CO, the single molecule adsorption energy of ethylene is smaller than that of the others. Repulsion between molecules in the adsorption process leads to a smaller co-adsorption energy and even physical adsorption, which influences the further reaction of acetate. Despite the co-adsorption energy of ethylene in the surface configuration, Pdc decreases significantly and Pds decreases slightly in the surface configuration and this energy is still far below the adsorption energy of CO and acetate. These results indicate that ethylene in a non-continuous Pd configuration surface may adsorb around acetate and further react with acetate. However, CO, due to its smaller molecular structure and larger adsorption energy, occupies more active Pd sites when the amount of CO increases, which significantly hinders the adsorption of ethylene, resulting in decreased catalytic activity, and even loss of activity.
3.6 CO + Oa → CO2
Considering that there is CO with ethylene in the upper stream products and CO as micro-molecules can easily adsorb on the surfaces of the metal catalysts, we have found that the adsorption energy of CO is larger than that of the ethylene in the vinyl acetate production system. Therefore, it will impact the ethylene adsorption and then suppress the reaction of acetate with ethylene when CO occupies the active site. Then, we considered where CO could be present in the reaction system and found it necessary to demonstrate the complexity of CO to CO2 catalyzed by Pd–Au with the DFT method.
3.6.1 Elementary reaction of CO and Oa. With the stable co-adsorption structure of CO and Oa as the initial reactants (Initial State, IS), the stable adsorption structure of CO2 as the final product (Final State, FS), structure parameters of the transition state, energy of the reaction barrier and adsorption energy of CO2 in the elementary steps of CO oxidation were calculated. The following figure shows the structure of the initial state (IS), transition state (TS), and final state (FS) at different configuration surfaces.From the structure shown in Fig. 12, in the reaction of CO and Oa atom with different configuration surfaces, the Oa atom gets closer to CO, and the CO adsorbed vertically on the adsorbent position becomes inclined to the metal surface. Further, the distance of the C atom in CO and the Oa atom continuously reduces until CO and the Oa atom form the transition state structure of O–C–O*a.
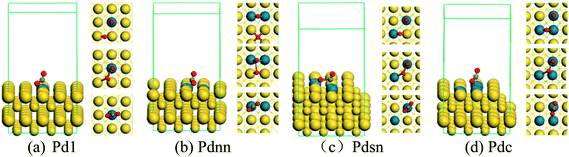 |
| Fig. 12 The geometry of IS, TS and FS in the CO oxidation reaction. | |
3.6.2 Transition state search. Fig. 13 shows the energy change in the process of CO oxidation, where IS stands for (CO + Oa)/Pd–Au in which CO and Oa exist in a co-adsorption system in the surface of different configurations, TS represents the transition state, and FS stands for CO2/Pd–Au in which CO2 exists in an adsorption system in surfaces having different configurations.
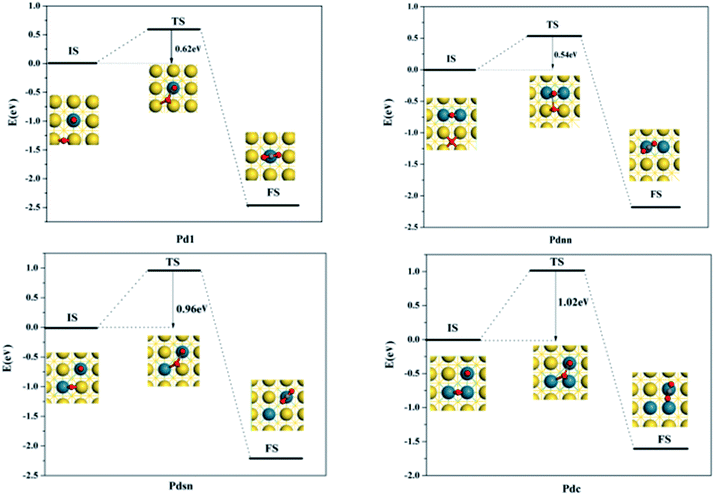 |
| Fig. 13 The energy profiles of the CO oxidation reaction. | |
From the data shown in Fig. 13 and 14, the energy barrier of CO oxidation is different in Pd1, Pdnn, Pdsn and Pdc, indicating that different configurations have different catalytic activity. The energy barrier of the Pdnn surface is the smallest for 0.54 eV, while the energy barrier of Pdc surface is the maximum for 1.02 eV. Further, other reaction energy barriers can be listed as follows: Pd1 (0.62 eV) and Pdsn (0.96 eV). These data illustrate that separate Pd monomer and continuous Pdnn geometries have high catalytic activity to the CO oxidation, which is consistent with the result of different configurations on Pd–Au(111) calculated by Yuan et al.23
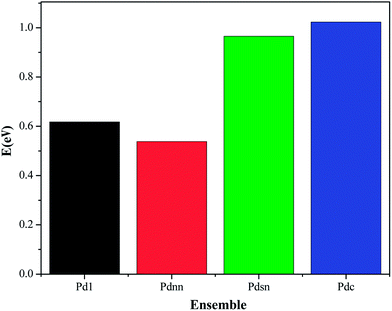 |
| Fig. 14 The energy barrier of CO oxidation on the Pd–Au(100) surface. | |
It can be seen that the energy barrier of elementary steps is not high and the adsorption energy of product CO2 in different configuration surfaces is close to 0.15 eV, which is physical adsorption and easy to remove on the surface. For the CO oxidation reaction, the Pd–Au catalyst has excellent catalytic activity, which is consistent with the conclusion of the experiments.24
4. Conclusion
In this work, the mono-adsorption properties of ethylene and acetate, co-adsorption of reactants with carbon monoxide on the Pd–Au(100) surface, and the transition state search of CO to CO2 have been systematically studied by using the DFT method. Calculation results indicate that the adsorption energy of CO is far higher than that of ethylene, and the adsorption of CO hinders that of ethylene with the amount of CO increasing, which affects the activity of the Pd catalyst; moreover, the Pd–Au catalyst has catalytic activity for CO oxidation reaction, but this catalytic activity can be influenced by the reaction rate and content of CO. Therefore, by controlling only the content of CO to a certain extent, we can achieve the conversion of CO to CO2 and reduce the effect of CO on the Pd catalyst activity.
References
- G. S. Grover and R. V. Chaudhari, Kinetics of oxidation of ethylene to vinyl acetate using a homogeneous palladium complex catalyst, Chem. Eng. J., 1986, 32, 93 CrossRef CAS.
- C. R. Reilly and J. J. Lerou, Supported liquid phase catalysis in selective oxidation, Catal. Today, 1998, 41, 433 CrossRef CAS.
- E. Balbolov and S. D. Dimitrov, Acetoxylation of (E,E,Z)-1,5,9-cyclododecatriene catalyzed by palladium(II) acetate in the presence of copper(II) acetate and air: A kinetic study, J. Mol. Catal. A: Chem., 1997, 126, 37–41 CrossRef CAS.
- D. D. Kragten, R. A. van Santen, M. K. Crawford, W. D. Provine and J. J. Lerou, A Spectroscopic Study of the Homogeneous Catalytic Conversion of Ethylene to Vinyl Acetate by Palladium Acetate, Inorg. Chem., 1999, 38, 331 CrossRef CAS.
- D. Stacchiola, F. Calaza, L. Burkholder, A. W. Schwabacher, M. Neurock and W. T. Tysoe, Elucidation of the reaction mechanism for the Pd-catalyzed synthesis of vinyl acetate, Angew. Chem., Int. Ed., 2005, 44, 4572–4574 CrossRef CAS PubMed.
- F. Calaza, D. Stacchiola, M. Neurock and W. T. Tysoe, Coverage Effects on the Pd-Catalyzed Synthesis of Vinyl Acetate: Comparison between Theory and Experiment, J. Am. Chem. Soc., 2002, 132, 2202–2207 CrossRef PubMed.
- E. Hansen and M. Neurock, First-principles based kinetic simulations of acetic acid temperature programmed reaction on Pd(111), J. Phys. Chem. B, 2001, 105, 9218–9229 CrossRef CAS.
- T. Owens, T. Jones and T. Noakes, et al., The effects of gold and Co-adsorbed carbon on the adsorption and thermal decomposition of acetic acid on Pd {111}, J. Phys. Chem. B, 2006, 110, 21152–21160 CrossRef CAS PubMed.
- Y. F. Han, J. H. Wang and D. Kumar, et al., A kinetic study of vinyl acetate synthesis over Pd-based catalysts: kinetics of vinyl acetate synthesis over Pd–Au/SiO2 and Pd/SiO2 catalysts, J. Catal., 2005, 232, 467–475 CrossRef CAS PubMed.
- Y. F. Han, D. Kumar and D. W. Goodman, Particle size effects in vinyl acetate synthesis over Pd/SiO2, J. Catal., 2005, 230, 353–358 CrossRef CAS PubMed.
- A. Morschbacker, Bio-Ethanol Based Ethylene, Polym. Rev., 2009, 49, 79–84 CrossRef CAS.
- M. S. Chen and D. W. Goodman, Promotional Effects of Au in Pd–Au Catalysts for Vinyl Acetate Synthesis, Chin. J. Catal., 2008, 29, 1178–1186 CrossRef CAS.
- M. S. Chen, D. Kumar and C. W. Yi, et al., The promotional effect of gold in catalysis by Pd–gold, Science, 2005, 310, 291–293 CrossRef CAS PubMed.
- F. Calaza, Z. Li and F. Gao, et al., The adsorption and reaction of vinyl acetate on Au/Pd(111) alloy surfaces, Surf. Sci., 2008, 602, 3523–3530 CrossRef CAS PubMed.
- T. Wei, D. Kumar and M. S. Chen, et al., Vinyl acetate synthesis over model PdSn bimetallic catalysts, J. Phys. Chem. C, 2008, 112, 8332–8337 CAS.
- B. Hammer, L. B. Hansen and J. K. Nørskov, Improved adsorption energetics within density-functional theory using revised Perdew–Burke–Ernzerhof functionals, Phys. Rev., 1999, 59, 413–7421 Search PubMed.
- H. J. Monkhorst and J. D. Pack, Special points for Brillouin-zone integrations, Phys. Rev., 1976, 13, 5188–5192 Search PubMed.
- T. A. Kirichenko, D. Yu, S. K. Banerjee and G. S. Hwang, Advanced Short-Time Thermal Processing for Si-Based CMOS Devices, The Electrochemical Society, Inc., 65 South Main Street Pennington, New Jersey 08534-2839, USA , 2004 Search PubMed.
- J. Kioseoglou, P. Komninou, J. Chen, G. Nouet, E. Kalesaki and T. Karakostas, Structural and electronic properties of elastically strained InN/GaN quantum well multilayer heterostructures Special points for Brillouin-zone integrations, Phys. Status Solidi C, 2014, 11(2), 289–292 CrossRef CAS.
- L. Árnadóttir, H. Jónsson and E. M. Stuve, The Effect of Co-adsorbed Water on the Stability and Configuration of Formyl (HCO) and Hydroxymethylidyne (COH) Intermediates on Pt(111): A Density Functional Theory Study, ECS Trans., 2008, 16(2), 621–626 Search PubMed.
- F. Calaza, F. Gao, Z. Li and W. T. Tysoe, The adsorption of ethylene on Au/Pd(111) alloy surfaces, Surf. Sci., 2007, 601, 714–722 CrossRef CAS PubMed.
- Q. Ge and M. Neurock, Correlation of adsorption energy with surface structure: ethylene adsorption on Pd surfaces, Chem. Phys. Lett., 2002, 358, 377–382 CrossRef CAS.
- D. W. Yuan, Z. R. Liu and J. H. Chen, Catalytic activity of Pd ensembles over Au(111) surface for CO oxidation: A first-principles study, J. Chem. Phys., 2011, 134, 054704 CrossRef CAS PubMed.
- F. Gao, Y. L. Wang and D. W. Goodman, CO Oxidation over AuPd(100) from Ultrahigh Vacuum to Near-Atmospheric Pressures: CO Adsorption-Induced Surface Segregation and Reaction Kinetics, J. Phys. Chem. C, 2009, 113, 14993–15000 CAS.
|
This journal is © The Royal Society of Chemistry 2014 |
Click here to see how this site uses Cookies. View our privacy policy here.