DOI:
10.1039/C4RA01077A
(Paper)
RSC Adv., 2014,
4, 17478-17482
Will a graphitic-like ZnO single-layer be an ideal substrate for graphene?
Received
11th January 2014
, Accepted 20th February 2014
First published on 21st February 2014
Abstract
The interaction between graphene and substrates may destroy the intrinsic properties of graphene, and reduce the potential applications of graphene in electronic devices. Here, we use first-principles calculations to explore the possibility of a graphitic ZnO layer as an ideal substrate for graphene. Taking graphitic ZnO with and without oxygen vacancies, we found that the intrinsic linear dispersion of graphene is well retained. Additionally, the resultant bilayer structure of graphene and the graphitic ZnO layer shows much better optical properties compared with separate graphene and graphitic ZnO. Moreover, we also found that both the band dispersion and Fermi velocity of the bilayer structured graphene are robust towards an external electric field. Therefore, our results indicate that a graphitic ZnO layer may be a suitable substrate for graphene in real applications.
1. Introduction
Graphene, which is composed of a single layer of carbon atoms arranged in a two-dimensional (2D) honeycomb lattice, has attracted great research interest. Because of its unique properties,1–3 graphene has been proposed as a potential material for next-generation devices, including high-speed electronic4 and optical devices,5 energy generation and storage,5–7 hybrid materials,8,9 chemical sensors,3,10 and so on. However, in practical applications of graphene, the typical substrates, such as SiO2,11–13 SiC,14–18 and some metal surfaces,19–22 form strong interactions with graphene, which destroy the intrinsic electronic states of graphene and significantly lower its carrier mobility. So it is quite interesting to find a suitable substrate which can better preserve the intrinsic properties of graphene.
Recently, there has been more and more work focusing on the heterostructures of graphene and other 2D crystals, such as graphene/graphitic born nitride,23–25 graphene/MoS2 nanosheets,26–28 graphene/MoSe2 nanosheets,29,30 graphene/graphitic carbon nitride,31,32 and graphitic ZnO (g-ZnO).33 Differing from traditional substrates consisting of thin films, such 2D crystal based heterostructures have shown many novel properties. Interestingly, most of these 2D crystals can preserve the high carrier mobility of graphene, and improve its optical properties.33 Consequently, such heterostructures hold great promise for future applications.
On the other hand, almost all of the studies focus on the perfect structures of 2D crystals, and the effects of defects are totally ignored. However, in any real application, defects cannot be fully avoided. For example, when the number of layers of ZnO (0001) film is reduced, it indeed prefers a graphitic honeycomb structure.34 However, oxygen vacancies (VO) are naturally abundant defects in ZnO. Therefore, the prepared graphitic ZnO (g-ZnO) may contain many oxygen vacancies. For the graphene/g-ZnO bilayer structure (GZO), how oxygen vacancies affect the electronic structure of graphene is still not clear. Since oxygen vacancies bring external carriers, it is natural to ask whether g-ZnO with oxygen vacancies (g-ZnOV) could destroy the high carrier mobility of graphene.
In the present work, we investigated the electronic structure and optical properties of GZO with and without oxygen vacancies via first-principles calculations. Our results indicate that graphene shows weak interactions with g-ZnO and g-ZnOV. Importantly, GZO with and without oxygen vacancies can better preserve graphene’s intrinsic electronic properties, high carrier mobility, and optical absorptions. Furthermore, the band dispersion and Fermi velocity of these hybrid structures are robust under an external electric field. According to our studies, g-ZnO may be a suitable substrate for graphene for practical applications in electronic and photoactive devices.
2. Computational method
Our first-principles calculations were based on density functional theory (DFT) with a generalized gradient approximation (GGA)35 for the exchange correlation potential. The Perdew–Burke–Ernzerholf (PBE) functional was used for the GGA as implemented in the Vienna ab initio simulation package (VASP).36 A van der Waals (vdW) correction proposed by Grimme (DFT-D2)37 was chosen due to its good description of long-range vdW interactions.38,39 The structures were relaxed without any symmetry constraints with a cutoff energy of 500 eV. Reciprocal space was represented by a Monkhorst–Pack special k-point scheme40 with 5 × 5 × 1 grid meshes. The convergence criteria of energy and force were set to 1 × 10−5 eV and 0.01 eV Å−1, respectively. A vacuum space consisting of a 17 Å normal to graphene plane was used to avoid interactions between two layers. The accuracy of our procedure was tested by calculating the C–C bond length of graphene; our calculated result of 1.407 Å is in good agreement with the experimental value of 1.420 Å.
The calculated unit cell lattice parameters for graphene and the g-ZnO monolayer were 2.46 and 3.25 Å, which fully agrees with previous experimental measurements and theoretical studies.41,42 We constructed a supercell consisting of 32 carbon atoms, 9 oxygen atoms and 9 zinc atoms as shown in Fig. 1(a). A 4 × 4 supercell of graphene was used to match a 3 × 3 supercell of the g-ZnO monolayer. The g-ZnO monolayer was assumed to be a substrate and we used a lattice constant similar to that of g-ZnO. The lattice mismatch was only 0.92%, which was almost the most suitable supercell. For GZOs with an oxygen vacancy (GZOV), we used the term GZOV-n (n = 1, 2, 3, 4, 5, 6) to denote composite structures with different VO positions, as shown in Fig. 1.
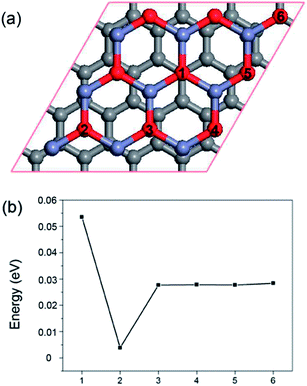 |
| Fig. 1 (a) Optimized structure of the GZO nanocomposite. The numbers 1–6 represent six possible positions for the oxygen vacancy, which are denoted as GZOV-n (n = 1–6). The gray, red, and blue balls denote carbon, oxygen, and zinc atoms, respectively. (b) Optimized energies correspond to different composite structures. The horizontal axis 1–6, represents GZOV-1 to GZOV-6. | |
3. Results and discussion
As shown in Fig. 1(a), there are six possible positions for the oxygen vacancy to be located according to symmetry arguments. To find the most energetically favorable structure, we freely relaxed all of the atoms without any restriction. By calculating the total energy of different configurations (as shown in Fig. 1(b)), we found that GZOV-2 is the most stable structure, while all the other structures are higher in energy by at least 25 meV. Therefore, the following discussions are mainly focused on the GZOV-2 structure.
First of all, the electronic properties of GZO and GZOV-2 were carefully investigated. It has been well documented that monolayered graphene shows a linear dispersion relationship E(k) = ±ħνF|k| (νF is the Fermi velocity) at around the Fermi level. For the GZO system, the linear dispersion character of graphene is well preserved, as shown in Fig. 2(a). The calculated νF of GZO is 4.89 × 105 m s−1, which agrees well with previous studies.33 On the other hand, for GZOV-2, it is quite surprising that the intrinsic linear dispersion of graphene is also well preserved (as shown in Fig. 2(b)), and the calculated νF of GZOV-2 is 4.82 × 105 m s−1, which is very close to that of free-standing graphene. Differing from hexagonal boron nitride (h-BN), both g-ZnO and g-ZnOV cannot widen the energy gap of graphene. By carefully looking at the calculated band structures of GZO and GZOV-2, we found that there were two important characteristics: (1) there was no chemical interaction between graphene and g-ZnO (g-ZnOV); and (2) the electronic states contributed by g-ZnO (g-ZnOV) were well separated with that of graphene.
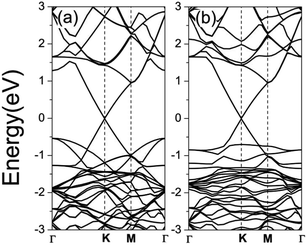 |
| Fig. 2 Electronic band structures of (a) GZO, and (b) GZOV. | |
To explore how the substrates affect the electronic properties of graphene, we plot differential charge densities (Δρ = ρ(GZO) − ρ(graphene) − ρ(g-ZnO)/ρ(g-ZnOV)) in Fig. 3. It should be noted that there is no charge transfer between graphene and substrates, because the electronic structure of graphene is well preserved. However, the inhomogeneous substrates indeed induce charge redistribution in the graphene plane, forming intralayer electron–hole puddles.43 Now, we can understand why the GZOV-2 structure is the most favored in energy. For g-ZnOV, most of the defective states are distributed around the oxygen vacancy. Thus, the relative stability of GZOV is dominated by the coulomb interaction between the defective states of g-ZnOV and the delocalized π states. Consequently, when the oxygen vacancy is located exactly in the middle of the hexagon of graphene, the coulomb interaction is the weakest, favoring the GZOV-2.
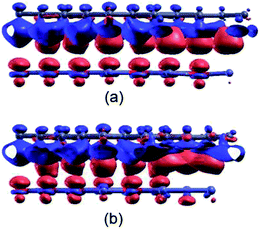 |
| Fig. 3 Differential charge density with an isosurface value of 0.002 e Å−3 for (a) GZO, and (b) GZOV. The red and blue regions indicate an increase and decrease in electron density, respectively. | |
Since GZO has shown excellent optical properties, it is interesting to study the optical properties of GZOV-2. As shown in Fig. 4, we plot the imaginary part of the dielectric functions. Although the absorption edges should have a rigid shift (of about 1.5 eV) due to the underestimation of the band gap in DFT calculations,44 it has been shown that the tendency of the calculated optical properties is reasonable.45–47 Compared with GZO, GZOV-2 exhibits similar a visible light response. More importantly, all of the other GZOV structures have similar optical properties. In other words, the positions of the oxygen vacancies do not change the optical adsorption. Therefore, g-ZnO can be used as an important substrate to enhance the optical properties of graphene, which may hold great potential in photocatalytic and photovoltaic applications.
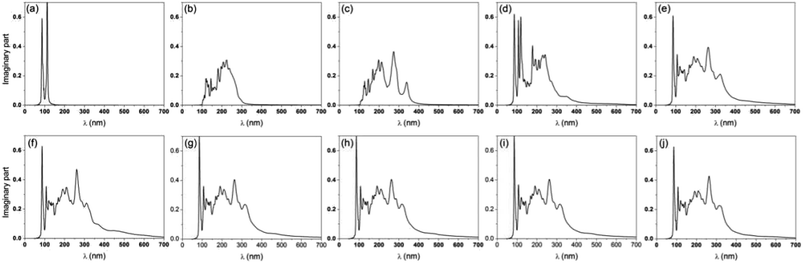 |
| Fig. 4 Imaginary part of the dielectric function of (a) graphene, (b) g-ZnO (c) g-ZnOV, (d) GZO, (e) GZOV-1, (f) GZOV-2, (g) GZOV-3, (h) GZOV-4, (i) GZOV-5, and (j) GZOV-6 for the polarization vector perpendicular to the surface. | |
Although we have shown that g-ZnO may be one of the most suitable substrates for graphene, it is not clear whether such a situation will be changed in real applications. As we know, an external electric field Eext is inevitable for practical applications in electronic devices. Therefore, it is quite interesting to explore the response of GZO and GZOV under an external electric field. To simulate the external electric field, a sawtooth like potential was applied along the direction perpendicular to the graphene plane, and the positive direction of the electric field was defined from the g-ZnO plane to the graphene plane. Fig. 5 shows the calculated band structures of GZO and GZOV-2 under an external electric field. It is quite clear that the linear dispersion relationship around the Dirac point is robust against an external electric field. For GZO, the occupied bands resulting from g-ZnO are quickly shifted in energy by the external electric field, and are almost degenerate with the Fermi level of graphene under an external electric field of 3 V nm−1. However, for GZOV-2, we can see that the relative shift in energy is much smaller than that for GZO.
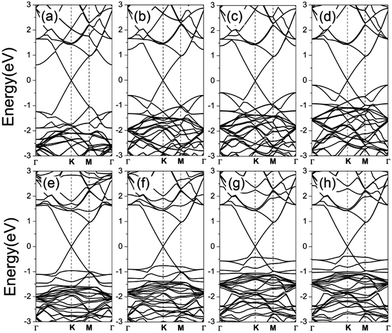 |
| Fig. 5 Electronic band structures of (a) GZO, Eext = −3 V nm−1, (b) GZO, Eext = −1 V nm−1, (c) GZO, Eext = 1 V nm−1, (d) GZO, Eext = 3 V nm−1, (e) GZOV-2, Eext = −3 V nm−1, (f) GZOV-2, Eext = −1 V nm−1, (g) GZOV-2, Eext = 1 V nm−1, and (h) GZOV-2, Eext = 3 V nm−1. The positive direction of Eext is from the g-ZnO plane to the graphene plane. | |
On the other hand, the mobility of the carriers is the most important characteristic of graphene, and is closely related to its potential applications. From electronic band calculations, we calculated the Fermi velocities of GZO and GZOV-2 under the applied external electric field. As shown in Fig. 6 the calculated νF of GZO decreased slightly when the field changed from −3 to 3 V nm−1, namely, νF changed from 4.91 × 105 m s−1 to 4.84 × 105 m s−1 with a small fluctuation of about 1.44%, which could be regarded as a constant. For GZOV-2, our calculated results showed that νF had the same tendency, and kept its high-speed character. For comparison, we also calculated the νF of all of the other GZOV structures, and found that νF was only changed by 2.27%, 1.95%, 1.83%, 1.87%, 2.18% for GZOV-1, GZOV-3, GZOV-4, GZOV-5, and GZOV-6, respectively. Obviously, νF in all of the GZOV structures gives rise to the high-speed character.
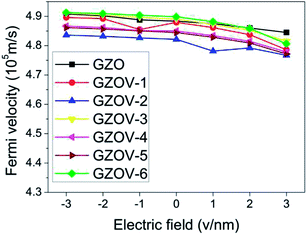 |
| Fig. 6 Variation of the Fermi velocities of GZO and GZOV-1 to GZOV-6 against external electric field. | |
Why doesn’t Eext destroy the high carrier mobility of graphene? To explore this issue, we plotted the differential charge density of GZOV-2, Eext = −3 V nm−1, in Fig. 7. It is worth noting that Eext does not lead to charge transfer between graphene and substrate, but induces dipole–dipole interactions to screen the external electric potential. So we come to the conclusion that the monolayered g-ZnO may be one of the most suitable substrates for graphene to preserve its intrinsic electronic properties, high carrier mobility under a wide range of external electric field regardless of oxygen vacancies, and positions of the vacancies.
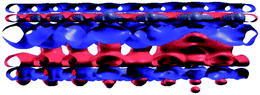 |
| Fig. 7 Differential charge density with an isosurface value of 0.002 e Å−3 for GZOV-2, Eext = −3 V nm−1. The positive direction of Eext is from the g-ZnO plane to the graphene plane. The red and blue regions indicate an increase and decrease in electron density, respectively. | |
4. Conclusions
In conclusion, we have theoretically demonstrated that a g-ZnO monolayer is a perfect substrate for graphene. Oxygen vacancies in g-ZnO do not have many unfavorable influences on the intrinsic electronic states of graphene, regardless of the positions of the vacancies. High carrier mobility can be preserved in both GZO and GZOV structures. Optically, oxygen vacancies do not destroy the enhanced visible light response of GZO more than simple graphene and g-ZnO monolayers. Furthermore, the band structures and Fermi velocities of GZO and GZOV are robust towards the external electric field for a wide range of field strengths.
Acknowledgements
This work was supported by the National Science Foundation of China (21203096, 11204137, 11247216, 11374160), the Natural Science Foundation of Jiangsu Province (BK20130031, BK2012392), the Fundamental Research Funds for the Central Universities (no. 30920130111016), New Century Excellent Talents in University (NCET-12-0628), Qinglan Project of Jiangsu Province, and NUST Research Funding (AB41374).
References
- K. S. Novoselov, A. K. Geim, S. V. Morozov, D. Jiang, Y. Zhang, S. V. Dubonos, I. V. Grigorieva and A. A. Firsov, Science, 2004, 306, 666–669 CrossRef CAS PubMed.
- K. S. Novoselov, A. K. Geim, S. V. Morozov, D. Jiang, M. I. Katsnelson, I. V. Grigorieva, S. V. Dubonos and A. A. Firsov, Nature, 2005, 438, 197–200 CrossRef CAS PubMed.
- A. K. Geim and K. S. Novoselov, Nat. Mater., 2007, 6, 183–191 CrossRef CAS PubMed.
- Y. M. Lin, C. Dimitrakopoulos, K. A. Jenkins, D. B. Farmer, H. Y. Chiu, A. Grill and P. Avouris, Science, 2010, 327, 662 CrossRef CAS PubMed.
- M. Liu, X. Yin, E. Ulin-Avila, B. Geng, T. Zentgraf, L. Ju, F. Wang and X. Zhang, Nature, 2011, 474, 64–67 CrossRef CAS PubMed.
- Y. Zhu, S. Murali, M. D. Stoller, K. J. Ganesh, W. Cai, P. J. Ferreira, A. Pirkle, R. M. Wallace, K. A. Cychosz, M. Thommes, D. Su, E. A. Stach and R. S. Ruoff, Science, 2011, 332, 1537–1541 CrossRef CAS PubMed.
- K. S. Kim, Y. Zhao, H. Jang, S. Y. Lee, J. M. Kim, K. S. Kim, J. H. Ahn, P. Kim, J. Y. Choi and B. H. Hong, Nature, 2009, 457, 706–710 CrossRef CAS PubMed.
- M. F. El-Kady, V. Strong, S. Dubin and R. B. Kaner, Science, 2012, 335, 1326–1330 CrossRef CAS PubMed.
- X. Yang, M. Xu, W. Qiu, X. Chen, M. Deng, J. Zhang, H. Iwai, E. Watanabe and H. Chen, J. Mater. Chem., 2011, 21, 8096–8103 RSC.
- M. Deng, X. Yang, M. Silke, W. M. Qiu, M. S. Xu, G. Borghs and H. Z. Chen, Sens. Actuators, B, 2011, 158, 176–184 CrossRef CAS PubMed.
- M. Ishigami, J. H. Chen, W. G. Cullen, M. S. Fuhrer and E. D. Williams, Nano Lett., 2007, 7, 1643–1648 CrossRef CAS PubMed.
- P. Shemella and S. K. Nayak, Appl. Phys. Lett., 2009, 94, 032101 CrossRef PubMed.
- T. C. Nguyen, M. Otani and S. Okada, Phys. Rev. Lett., 2011, 106, 106801 CrossRef.
- S. Y. Zhou, G. H. Gweon, A. V. Fedorov, P. N. First, W. A. de Heer, D. H. Lee, F. Guinea, A. H. Castro Neto and A. Lanzara, Nat. Mater., 2007, 6, 770–775 CrossRef CAS PubMed.
- A. Mattausch and O. Pankratov, Phys. Rev. Lett., 2007, 99, 076802 CrossRef.
- F. Varchon, R. Feng, J. Hass, X. Li, B. N. Nguyen, C. Naud, P. Mallet, J. Y. Veuillen, C. Berger, E. H. Conrad and L. Magaud, Phys. Rev. Lett., 2007, 99, 126805 CrossRef CAS.
- S. Kim, J. Ihm, H. J. Choi and Y. W. Son, Phys. Rev. Lett., 2008, 100, 176802 CrossRef.
- J. Ristein, S. Mammadov and T. Seyller, Phys. Rev. Lett., 2012, 108, 246104 CrossRef CAS.
- G. Giovannetti, P. A. Khomyakov, G. Brocks, V. M. Karpan, J. van den Brink and P. J. Kelly, Phys. Rev. Lett., 2008, 101, 026803 CrossRef CAS.
- P. A. Khomyakov, G. Giovannetti, P. C. Rusu, G. Brocks, J. van den Brink and P. J. Kelly, Phys. Rev. B: Condens. Matter Mater. Phys., 2009, 79, 195425 CrossRef.
- C. Gong, G. Lee, B. Shan, E. M. Vogel, R. M. Wallace and K. Cho, J. Appl. Phys., 2010, 108, 123711 CrossRef PubMed.
- M. Vanin, J. J. Mortensen, A. K. Kelkkanen, J. M. Garcia-Lastra, K. S. Thygesen and K. W. Jacobsen, Phys. Rev. B: Condens. Matter Mater. Phys., 2010, 81, 081408 CrossRef.
- G. Giovannetti, P. A. Khomyakov, G. Brocks, P. J. Kelly and J. van den Brink, Phys. Rev. B: Condens. Matter Mater. Phys., 2007, 76, 073103 CrossRef.
- C. R. Dean, A. F. Young, I. Meric, C. Lee, L. Wang, S. Sorgenfrei, K. Watanabe, T. Taniguchi, P. Kim, K. L. Shepard and J. Hone, Nat. Nanotechnol., 2010, 5, 722–726 CrossRef CAS PubMed.
- Y. C. Fan, M. W. Zhao, Z. H. Wang, X. J. Zhang and H. Y. Zhang, Appl. Phys. Lett., 2011, 98, 083103–083103 CrossRef PubMed.
- K. Chang and W. Chen, Chem. Commun., 2011, 47, 4252–4254 RSC.
- Y. Ma, Y. Dai, M. Guo, C. Niu and B. Huang, Nanoscale, 2011, 3, 3883–3887 RSC.
- L. Britnell, R. V. Gorbachev, R. Jalil, B. D. Belle, F. Schedin, A. Mishchenko, T. Georgiou, M. I. Katsnelson, L. Eaves, S. V. Morozov, N. M. R. Peres, J. Leist, A. K. Geim, K. S. Novoselov and L. A. Ponomarenko, Science, 2012, 335, 947–950 CrossRef CAS PubMed.
- J. N. Coleman, M. Lotya, A. O'Neill, S. D. Bergin, P. J. King, U. Khan, K. Young, A. Gaucher, S. De, R. J. Smith, I. V. Shvets, S. K. Arora, G. Stanton, H.-Y. Kim, K. Lee, G. T. Kim, G. S. Duesberg, T. Hallam, J. J. Boland, J. J. Wang, J. F. Donegan, J. C. Grunlan, G. Moriarty, A. Shmeliov, R. J. Nicholls, J. M. Perkins, E. M. Grieveson, K. Theuwissen, D. W. McComb, P. D. Nellist and V. Nicolosi, Science, 2011, 331, 568–571 CrossRef CAS PubMed.
- Y. Ma, Y. Dai, W. Wei, C. Niu, L. Yu and B. Huang, J. Phys. Chem. C, 2011, 115, 20237–20241 CAS.
- Q. Xiang, J. Yu and M. Jaroniec, J. Phys. Chem. C, 2011, 115, 7355–7363 CAS.
- A. Du, S. Sanvito, Z. Li, D. Wang, Y. Jiao, T. Liao, Q. Sun, Y. H. Ng, Z. Zhu, R. Amal and S. C. Smith, J. Am. Chem. Soc., 2012, 134, 4393–4397 CrossRef CAS PubMed.
- W. Hu, Z. Li and J. Yang, J. Chem. Phys., 2013, 138, 124706 CrossRef PubMed.
- C. Tusche, H. L. Meyerheim and J. Kirschner, Phys. Rev. Lett., 2007, 99, 026102 CrossRef CAS.
- J. P. Perdew, K. Burke and M. Ernzerhof, Phys. Rev. Lett., 1996, 77, 3865–3868 CrossRef CAS.
- G. Kresse and J. Furthmüller, Phys. Rev. B: Condens. Matter Mater. Phys., 1996, 54, 11169–11186 CrossRef CAS.
- S. Grimme, J. Comput. Chem., 2006, 27, 1787–1799 CrossRef CAS PubMed.
- S. Grimme, C. Mück-Lichtenfeld and J. Antony, J. Phys. Chem. C, 2007, 111, 11199–11207 CAS.
- V. Caciuc, N. Atodiresei, M. Callsen, P. Lazić and S. Blüge, J. Phys.: Condens. Matter, 2012, 24, 424214 CrossRef PubMed.
- H. J. Monkhorst and J. D. Pack, Phys. Rev. B: Solid State, 1976, 13, 5188–5192 CrossRef.
- A. H. Castro Neto, F. Guinea, N. M. R. Peres, K. S. Novoselov and A. K. Geim, Rev. Mod. Phys., 2009, 81, 109–162 CrossRef CAS.
- H. Y. Guo, Y. Zhao, N. Lu, E. J. Kan, X. C. Zeng, X. J. Wu and J. L. Yang, J. Phys. Chem. C, 2012, 116, 11336–11342 CAS.
- J. Martin, N. Akerman, G. Ulbricht, T. Lohmann, J. Smet, K. Von Klitzing and A. Yacoby, Nat. Phys., 2007, 4, 144–148 CrossRef.
- S. Lany and A. Zunger, Phys. Rev. B: Condens. Matter Mater. Phys., 2008, 78, 235104 CrossRef.
- A. Du, Y. H. Ng, N. J. Bell, Z. Zhu, R. Amal and S. C. Smith, J. Phys. Chem. Lett., 2011, 2, 894–899 CrossRef CAS.
- F. Wu, Y. Liu, G. Yu, D. Shen, Y. Wang and E. Kan, J. Phys. Chem. Lett., 2012, 3, 3330–3334 CrossRef CAS.
- J.-H. Yang, Y. Zhai, H. Liu, H. Xiang, X. Gong and S.-H. Wei, J. Am. Chem. Soc., 2012, 134, 12653–12657 CrossRef CAS PubMed.
|
This journal is © The Royal Society of Chemistry 2014 |
Click here to see how this site uses Cookies. View our privacy policy here.