A novel fluffy hydroxylapatite fiber scaffold with deep interconnected pores designed for three-dimensional cell culture†
Received
3rd September 2013
, Accepted 24th October 2013
First published on 28th October 2013
Abstract
Functional scaffolds that could mimic cells' natural growth state in vitro are crucial for meeting the requirements of complex biological systems. However, the compact macrostructure and poor cell survival macro-environment of biomaterials are still two major challenges limiting their practical applications in bone tissue regeneration. In this study, we fabricated a highly fluffy and porous biomineral hydroxylapatite (HA) encapsulated poly-L-lactic acid (PLLA) composite fibrous scaffold (fluffy-HAFSs) by employing an improved electrospinning technique combined with a bio-mineralization technique. In the scaffold, deep interconnected pores of 65 ± 35 μm formed among these fluffy HA fibers, which permitted the easy entry of cells into the fluffy-HAFSs with no extra help to achieve complicated 3D cell culture methodologies. Human mesenchymal stem cells (hMSCs) were seeded onto the composite fibrous scaffolds and cultured for 14 days in vitro. The morphology and biochemical activities of hMSCs were tested over the culture period. Evidence was provided for the hMSCs entry into the interior of the fluffy-HAFSs and achievement of 3D cell distribution. Furthermore, these hMSCs exhibited higher degrees of growth, osteogenic differentiation and mineralization than those on HA deposited traditional electrospun fibrous meshes (HAFMs). These results indicated that the novel fluffy-HAFSs might be potentially applied as bone repairing and regeneration scaffolds.
Introduction
In the past decades, considerable attention has been focused on preparation of various biomaterials for bone repairing and regeneration for their biocompatibility and suitable mechanical properties.1–7 For example, functional particles,8 mats,9–14 and three dimensional (3D) scaffolds15 have been developed and used in bone tissue engineering by incorporation into or coating onto inorganic bioactive fillers to impart cell activity.16 In particular, 3D biomaterials have received increasing attention due to their porous structure which provides sufficient space for cells' uniform distribution and facile delivery of oxygen and nutrients.17–22 More importantly, these 3D biomaterials partly mimic the topology and biological functions of the extracellular matrix (ECM) for guided bone repair and regeneration.23–26
As one of the important bone filler materials, hydroxyapatite (HA) has been widely used to fabricate various bone repairing materials due to its similar composition to the inorganic component of natural bone.27–30 Moreover, it has excellent bioactivity and biocompatibility, for example, it can provide a favorable environment for osteoconduction, protein adhesion, and osteoblast proliferation.31 In recent years, a large amount of composite scaffolds, such as PLA/HA,32 gelatin/HA,33,34 triphasic HA/collagen/PCL,35 PCL–PEG–PCL/HA,27etc., have been developed by incorporating HA for bone repairing and regeneration applications. The major characteristics of suitable 3D HA based bone repairing biomaterials are porous macrostructure and high HA content. In addition, these scaffolds should provide cell loading performance and excellent osteoconductivity. However, these current HA based bone repairing materials are far from fully meeting the performance requirements of ECM because of their low HA content and poor compact internal architecture, which are extremely unfavorable for achieving 3D cell culture. Although significant efforts have been made to develop new methods to obtain porous structural characteristics of HA based 3D scaffolds,36–39 the desirable bicontinuous pores in these scaffolds often disappeared during the bio-mineralization process. These drawbacks greatly limited the practical application of these HA based bone repairing materials.
The aim of the study is to develop a novel fluffy HA fibrous scaffold with interconnected pores for bone tissue engineering. Firstly, an optimized electrospinning technique (as shown in Fig. 1A) was developed to prepare fluffy PLLA fibrous scaffolds (fluffy-FSs), in which the PLLA fibers were in the discrete state as shown in Fig. 1B-I. Subsequently, the bio-mineralization process was employed to obtain HA coating on the surface of the PLLA fibers (as shown in Fig. 1B-III). In the finally formed fluffy-HAFSs, numerous interconnected pores appeared which could permit easy entry of cells into the fluffy-HAFSs to achieve 3D cell culture. The effect of fluffy structure with interconnected pores in the scaffold on hMSCs was observed over a period of 14 days in culture, and assessed in terms of cell proliferation, morphology and functional expression compared to that on HA fibrous meshes (HAFMs) to evaluate the potential applications of this type of fluffy HA based scaffold in bone tissue engineering.
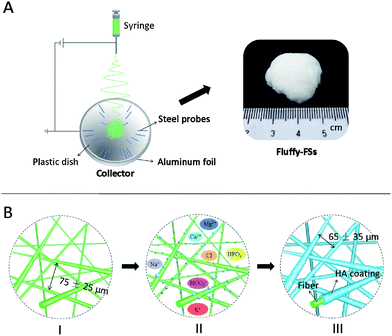 |
| Fig. 1 Illustration of the fabrication process for fluffy-HAFSs. (A) The fluffy-FSs were prepared by an improved electrospinning technique with a special hemispherical collector; (B-I) the PLLA fibers in the fluffy-FSs were discrete from one another with deep interconnected pores of 75 ± 25 μm, and then (B-II) the obtained fluffy-FSs were immersed in SBF solution, at the beginning of incubation some sporadic HA particles formed on the surface of PLLA fibers, (B-III) after 21 days incubation, these HA particles grew into a dense bio-mineral HA coating which encapsulated the entire surface of PLLA fibers, and the formed HA fibers in fluffy-HAFSs remained in the discrete state with deep interconnected pores of 65 ± 35 μm. | |
Materials and methods
Materials
PLLA (MW = 200 kDa) was obtained from Daigang Polymer (Jinan, China). All other chemicals were purchased from Sigma-Aldrich and used as received without further purification.
Fabrication of fluffy-FSs
The electrospinning process was employed to fabricate the PLLA nanofibers in this study. Briefly, 6.8 wt% PLLA solution dissolved in a mixed solution of dichloromethane–N,N-dimethyl-formamide (v/v = 9/1) was fed into a syringe capped with a 0.22 gauge blunt-tipped needle nozzle. And then, the solution was driven by using a syringe pump (Silugao Co., Beijing, China) at a speed of 1.5–2.5 mL h−1 with a voltage of 14 kV supplied by a high DC power supply (Dongwen High voltage, China). The distance between the tip of the needle and the top of the collector was ∼10 cm. The fluffy-FSs were obtained using a specially designed collector (Fig. 1A and S1 in the ESI†), which was crafted by embedding an array of 1.5 cm long stainless steel probes in a hemispherical plastic dish (diameter: 8 cm, shell thickness: 0.2 cm) covered with aluminum foil and backed by a stainless steel lining to provide an electrical ground, and the needles were placed at 2 cm intervals radiating from the center of the dish in 4–8 equidistant directions. These probes in the collector were able to collect the nanofibers from 4–8 equidistant directions, and allowed nanofiber layers to settle next to the previously deposited layers, finally forming the fluffy structure PLLA fibrous scaffold in the center of the hemispherical plastic dish (Fig. S2A in the ESI†). Then the fluffy-FSs were removed with a plastic rod slightly and kept in a vacuum dryer for further use (Fig. S2B in the ESI†).
Fabrication of fluffy-HAFSs and HAFMs
The simulated body fluid (SBF, pH = 7.2) used for the bio-mineralization was prepared as previously reported.24 Firstly, 0.01 g fluffy-FSs were immersed in a glass bottle (diameter of 3.5 cm) containing 50 mL 30% ethanol solution and shaken for 2 min for thorough infiltration and swelling; then the ethanol solution was removed and these scaffolds were washed gently with SBF several times to remove the ethanol completely. Subsequently, 50 mL fresh SBF were added into the above glass bottles and these were maintained at 37 °C to make these scaffolds remain in the mineralized state. The SBF was changed every other day. After being incubated for various time points, the SBF was removed from the bottles and these were filled with DI water several times to remove the soluble inorganic ions completely, and then these fluffy-HAFSs kept in the glass bottles were finally dried by freeze-drying for further study. The HAFMs were prepared by using PLLA fibrous meshes as templates in the above mineralization process.
Characterization of different scaffolds
The SEM images and X-ray energy dispersive spectrum (EDS) of different scaffolds were obtained on a Hitachi S-4800N at an acceleration voltage of 20 kV. Similar to the previous study, the Ca/P atomic ratio of HAFMs and fluffy-HAFSs was determined by EDS and RONTEC software.45 In order to measure the size and interconnectivity of the pores in the fluffy scaffolds, the cross-sections and longitudinal sections of these fluffy scaffolds were fabricated by cutting frozen shaped samples with a thickness of 2 mm, and then freeze-drying for SEM detection. In the SEM images, the pores in these scaffolds were defined as closed circles composed of fibers at the same level. The average pore size was obtained by measuring 100 different pores in the obtained SEM images by ImageJ software (NIH, USA). The FTIR spectra were recorded with a VERTEX 70 (Bruker Co. Germany) FTIR spectrometer. N2 adsorption measurements were carried out using a Micromeritics ASAP 2010 analyzer at 77 K. Mechanical properties of the scaffolds were measured using a micro-tensile testing machine (Sans-GB T528, ShenZhen, China). The average diameter and diameter distribution of these HA fibers and PLLA fibers in the scaffolds were determined by measuring diameters of 100 different fibers in a 645 × 484 (pixel) SEM image with ImageJ software. The water absorption rate was calculated by the quality of water absorbed/the quality of scaffolds.
hMSCs isolation
hMSCs were isolated as previously described from human bone marrow donations.40 Briefly, bone marrow aspirates were collected and centrifuged, then the cell pellet was resuspended in a complete medium (DMEM) (Hyclone, Logan, Utah) supplemented with 10% fetal bovine serum (FBS), and cultured in a 5% CO2 atmosphere at 37 °C. The adherent cells were allowed to reach approximately 80% confluence (7–9 days for the first passage). Cells were passaged during culture, and passage 3 cells (P3–5) were used for experiments.
Cell seeding
The obtained fluffy-HAFSs kept in the above glass bottles with a diameter of 3.5 cm was filled with 30 mL DI water and kept at −20 °C for shaping, and then heated using an alcohol lamp to break the glass bottles and the shaped fluffy-HAFSs were quickly moved into a cryostat microtome (Minotome, IEC, USA) at −5 °C; subsequently, the shaped fluffy-HAFSs were cut into thin sections with a thickness of 2.0 mm using a sterile razor carefully and finally dried by freeze-drying for cell seeding. The sterilization was done by soaking the scaffolds in a solution of 70% ethanol and 30% PBS for 12 h, followed by washing several times with PBS and then placing in 6-well plates. Afterwards, the scaffolds were washed with a complete medium (DMEM, 10% FBS, 100 U mL−1 penicillin and 100 mg mL−1 streptomycin) twice for 2 h each time on an orbital shaker (Lab-Line Instruments, Inc.). hMSCs were then seeded onto each of the fluffy-HAFSs (thickness of 2.0 mm) and HAFMs at a cell density of 1.0 × 104 cells per scaffold. The resulting scaffold/cell constructs were placed in an incubator for 6 h to allow for cell attachment, with an additional 3 mL of the appropriate medium added into each well afterwards. Growth medium consisting of DMEM supplemented with 10% FBS was used for studying cell morphology, attachment and proliferation. Osteogenic medium consisting of a-DMEM, 10% FBS, 0.1 μM dexamethasone (Sigma-Aldrich, MO, USA), 10 μM β-glycerophosphate (Calbiochem, CA, USA) and 50 μM ascorbic acid (Sigma-Aldrich) was used for the osteogenic differentiation studies. The medium was changed every day.
Cell proliferation
The cells in HAFMs and fluffy-HAFSs were quantified at various time points based on a DNA analysis method.41 Briefly, the adhered cells were lysed by immersion of the scaffold in 0.5% Triton X-100 DNA-free DI water and incubation at 37 °C for 1 h. The DNA concentration was quantified using a PicoGreens dsDNA quantification kit (Quant-iT Picogreen, P7589, Invitrogen) according to the manufacturer's protocol. Fluorescence was measured at an excitation wavelength of 485 nm and an emission wavelength of 530 nm using a plate reader. Culture medium soaked empty scaffolds subjected to the same processes were used as blank controls. Cell proliferation was expressed as the ratio of adhered cells to original seeded cells.
Cell fluorescence detection
To assess the cell viability, samples were incubated with 1 mL 5 μg mL−1 fluorescein diacetate (FDA, green, Sigma-Aldrich) in culture medium for 10 min at 37 °C, and then washed twice using PBS solution. In order to observe the hMSCs cultured in the interior of fluffy-HAFSs, the samples were cut into pieces by the ultrathin sectioning technique and imaged under a fluorescence microscope (Olympus, Japan).
Cell morphology
The cell morphology was visualized using a SEM. The cells were fixed with 3% glutaraldehyde for 2 h and dehydrated using incremental concentrations of ethanol–water (25%, 50%, 70%, 80%, 90%, 95% and absolute ethanol for 30 min each); subsequently they were dried by CO2 critical point drying (Hitachi HCP-2) and sputter-coated with platinum before observing under the SEM.
Alkaline phosphatase enzyme (ALP) activity
The ALP activity was determined by osteogenic differentiation using a LabAssay™ ALP assay kit (Genmed, China); each construct was measured at day 3, 7 and 14. At each time point, all constructs were rinsed with PBS three times, and lysed with 0.1% Triton X-100 solution. The ALP activity of the lysates was determined using p-nitrophenyl phosphate as a substrate. The absorbance was measured at 405 nm using a microplate reader.
Alizarin red S staining
Alizarin red S staining was used to evaluate hMSC mineralization on different scaffolds. Briefly at day 14, the samples were fixed in 4% paraformaldehyde at 4 °C for 24 h and washed three times with 0.01 M PBS and stained with the Alizarin Red solution (2%, pH 4.2) for 10 min, and subsequently they were washed several times with DI water to remove the remaining stain. Subsequently, the samples were imaged using a fluorescence microscope.
Statistical analysis
Data were expressed as the mean ± standard deviation. Statistical analyses were performed using the t-test. All data were analyzed with SPSS software (version 11.0).
Results and discussion
Fabrication of fluffy-HAFSs
We successfully fabricated fluffy-FSs (Fig. 1A) by the optimized electrospinning technique. Fig. 2C shows the morphology of the obtained PLLA fibers in the fluffy-FSs. Compared to the traditional electrospun PLLA fibrous meshes (FMs) (Fig. 2A), the majority of PLLA fibers in fluffy-FSs were discrete from one another, and the distance between them was about 75 ± 25 μm, a few PLLA fibers occupied at the same level. The unique structures made the fluffy-FSs very soft, which felt like a cloud of cotton. The PLLA fibers in the fluffy-FSs exhibited a narrow fiber size distribution from 1.6–2.8 μm with an average diameter of 2.15 μm (Fig. 3A). Here, we used the fluffy-FSs as templates to fabricate the fluffy-HAFSs by bio-mineralization. As shown in Fig. 2D, a thick and dense HA coating formed on the surface of PLLA fibers after 21 days of incubation in SBF, which encapsulated entire PLLA fibers. The obtained fluffy-HAFSs exhibited similar morphology to fluffy-FSs. In the fluffy-HAFSs, most of the HA fibers remained in the discrete state, only a few fibers intertwined with each other due to the growth of HA coating. These structural features led to numerous deep interconnected pores of 65 ± 35 μm among these HA fibers in the fluffy-HAFSs, which were large enough for cells (∼15 μm) to migrate into the deep center of the fluffy-HAFSs for 3D cell culture. We observed that the range of diameters of the HA fibers was from 8 μm to 14 μm, with an average diameter of 10.45 μm (Fig. 3B), which indicated that the thickness of the HA coating was ∼8.3 μm. In the study, we set the traditional FMs as a control to evaluate the effect of bio-mineralization of HA on the spatial structure of PLLA scaffolds. Fig. 2B shows the morphology of HAFMs; the pores in the original PLLA FMs (Fig. 2A) were filled by HA coating almost completely after 21 days of incubation in SBF, only a few pores with a size of ∼1 μm appeared on the surface of the HAFMs. Obviously, it was impossible for the cells to migrate into the interior of the HAFMs to achieve a true 3D cell culture, which could only form a cell monolayer or a cluster.
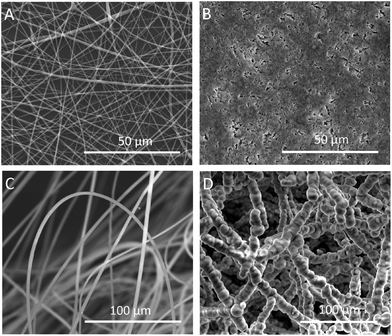 |
| Fig. 2 SEM images of FMs and fluffy-FSs before and after bio-mineralization of HA: (A) PLLA FMs, (B) HAFMs, (C) fluffy-FSs, and (D) fluffy-HAFSs. | |
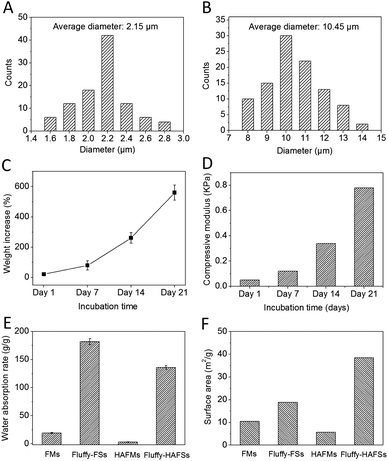 |
| Fig. 3 Size distribution of fibers in fluffy-FSs (A) and fluffy-HAFSs (B); mass increase (C) and compressive modulus (D) of fluffy-FSs after incubation in SBF at different time points; the water absorption rate (E) and surface area (F) of different scaffolds. Data are mean ± SD, n = 4. **p < 0.01. | |
Bio-mineralization process of HA
The HA coating resulted in a continually increased weight of fluffy-HAFSs (Fig. 3C); at day 21 in incubation the weight of fluffy-HAFSs was 6 fold greater than that of original fluffy-FSs, which indicated that the content of HA in fluffy-HAFSs was significantly higher than that of traditional HA based composite scaffolds.42 Additionally, the HA coating also led to a significantly enhanced mechanical strength (Fig. 3D) due to increasing interfacial adhesion between HA and PLLA with the incubation time, while the fluffy-HAFSs became hard and brittle compared to fluffy-FSs. At day 21, the compressive modulus of fluffy-HAFSs was up to 0.78 kPa (compressive ratio was 30%), much larger than that of original fluffy-FSs (4.2 Pa), which was strong enough as substrate materials to support cells 3D culture, and it was acceptable as filler materials for bone tissue engineering. The water absorption rate clearly reflected the porosity of different scaffolds as shown in Fig. 3E. The fluffy spatial structure of fluffy-FSs and fluffy-HAFSs led to a higher porosity compared to traditional FMs and HAFMs, respectively. The fluffy-HAFSs had slightly lower porosity than the fluffy-FSs because of the increased diameter of fibers from original ∼2.15 μm (PLLA fibers) to final ∼10.45 μm (HA fibers). However, the HA coating resulted in a higher surface area of fluffy-HAFSs (38.5 m2 g−1) than that of fluffy-FSs (18.8 m2 g−1) as shown in Fig. 3F, which would provide more area and space to cells for cell attachment and growth.
The formation of the HA coating on the surface of PLLA fibers in fluffy-FSs was observed clearly by examining the SEM images as shown in Fig. 4. After incubation in SBF for 7 days, a few of micro-particles with the size of 3–10 μm started to appear on the surface of PLLA fibers (Fig. 4A and B). Subsequently, a large number of HA particles formed along the PLLA fibers, and these particles adhered to each other loosely around the fibers (Fig. 4C and D). Finally, these HA particles grew into a thick and dense HA coating after 21 days of incubation in SBF (Fig. 4E and F). During the entire period of bio-mineralization of HA, the original fluffy spatial structure with deep interconnected pores of fluffy-FSs was maintained completely because of the slow and regular formation of HA coating on the surface of PLLA fibers in a static environment.
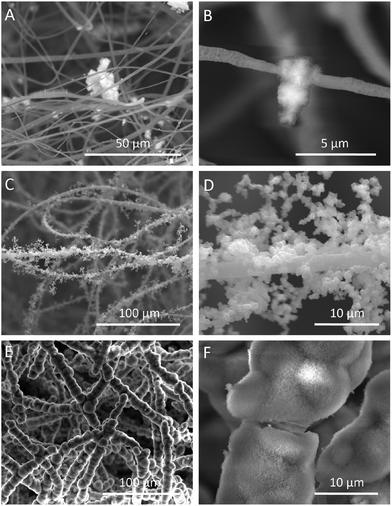 |
| Fig. 4 SEM images of the fluffy-FSs after incubation in SBF at different time points. (A and B) day 7; (C and D) day 14; (E and F) day 21. | |
Chemical characterization
The chemical characteristics of bio-mineral HA on the surface of PLLA fibers were observed by FTIR and EDS. As shown in Fig. 5A, the existence of HA was confirmed from FTIR spectra. Three peaks at 536, 604 and 1033 cm−1 are attributed to the vibrations in P–O bonds of phosphate groups from HA. The second peak is located at 1012 cm−1 in the pristine HA spectrum and shifted to a higher wavenumber (1033 cm−1) after HA coating. Characteristic peaks of PLLA (1750 cm−1 for the C
O group and at 1083 for C–O stretching) became weaker but exhibited no change in wavenumber in the fluffy-HAFS spectrum. These results were in accordance with the previous study. In addition, the EDS spectrum (Fig. 5B) showed that the main elements of the fluffy-HAFSs were oxygen, calcium and phosphorus and the Ca/P ratio was 1.60, indicating that the mineral phase is HA. These chemical characteristics of HA coating were in accordance with previous studies,43–45 which showed a successful bio-mineralization of HA on the surface of PLLA fibers in the fluffy-FSs.
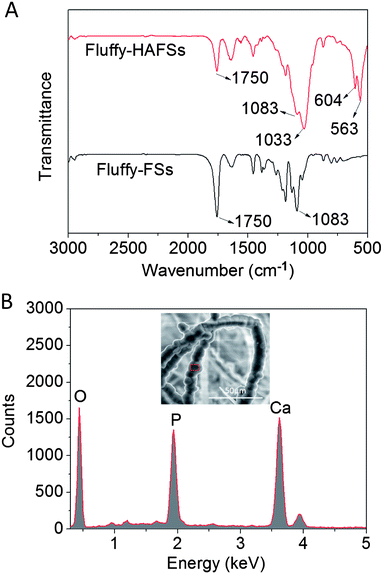 |
| Fig. 5 (A) FTIR spectra of fluffy-FSs and fluffy-HAFSs; (B) EDS spectrum and SEM image of fluffy-HAFSs. | |
Cells proliferation of hMSCs
In order to evaluate the effect of the fluffy spatial structure of fluff-HAFSs on the cell culture, the fluffy-HAFSs were cut into sections with a thickness of 2 mm for cell seeding. It was noteworthy that the operation of cutting must be slow and gradual because a fast cutting during the sectioning process would lead to shedding of small debris of HA coating. The HAFM was set as a control. The hMSCs were cultured on different scaffolds for a period of 7 days. Cell attachment and proliferation on these scaffolds were analyzed with the PicoGreen dsDNA assay. As shown in Fig. 6, after 12 h of culture, hMSCs exhibited similar cell attachment: 83% hMSCs adhered onto HAFMs compared to 81% of the hMSCs adhered onto the fluffy-HAFSs. During the culture period of 7 days, cell proliferation of hMSCs in fluffy-HAFSs is significantly higher than that of hMSCs in HAFMs. At day 7 in culture, the number of hMSCs in fluffy-HAFSs increased to 10.39 × 104 cells, while the number of hMSCs on HAFMs was 6.63 × 104 cells. These results strongly supported that the fluffy spatial structure with deep interconnected pores of fluffy-HAFSs could promote cell proliferation compared to traditional HAFMs.
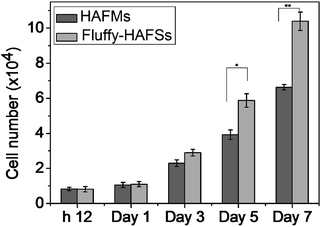 |
| Fig. 6 The attachment and proliferation of hMSCs cultured on different scaffolds in various incubation periods by the PicoGreen dsDNA assay. hMSCs were originally seeded at 1.0 × 104 cells. Data are mean ± SD, n = 4, *p < 0.05, and **p < 0.01. | |
Fluorescence detection and the morphology of hMSC
In order to examine the cell activity on different scaffolds, the hMSCs were stained with FDA after 7 days of culture. As shown in Fig. 7B, a large number of hMSCs appeared in the interior of fluffy-HAFSs and these hMSCs grew into cell clusters with high cell activity. In contrast, the hMSCs cultured on HAFMs displayed a separate shape with sparse cell–cell contact (Fig. 7A). The microscopic morphology of hMSCs cultured on the HAFMs and fluffy-HAFSs was examined by SEM after 7 days of culture. Fig. 7D shows that hMSCs in the fluffy-HAFSs formed integrated cell–fiber constructs and the cell membrane spread widely so that it was difficult to distinguish the morphology of single hMSCs. The cells in these constructs exhibited wide cell–cell contact, which was helpful for the maintenance of cell activity. In contrast, hMSCs cultured on the HAFMs maintained separate shapes with sparse cell–cell contact (Fig. 7C), which was in agreement with the results of the fluorescence images. These results indicated that the fluffy spatial structure ensured easy migration of cells into the interior of the fluffy-HAFSs to facilitate the 3D culture of hMSCs, improved the cell activity obviously and impacted the cell growth morphology.
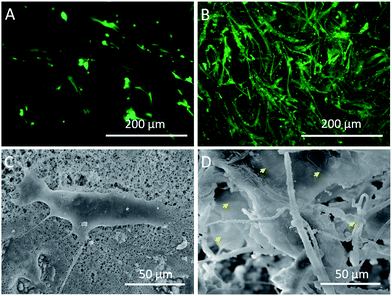 |
| Fig. 7 Fluorescence and SEM images of hMSCs cultured on different scaffolds at day 7: (A and C) HAFMs and (B and D) fluffy-HAFSs cells. Yellow arrows: spread cell membrane of hMSCs. | |
Alkaline phosphatase enzyme (ALP) activity and Alizarin red S staining
Cell osteogenic differentiation was assessed by determining the ALP activity of hMSCs cultured on HAFMs and fluffy-HAFSs respectively at 3, 7 and 14 days of culture. As shown in Fig. 8A, the ALP activity of hMSCs expressed low and similar levels at day 3 on HAFMs and fluffy-HAFSs. However, after 7 and 14 days of incubation, the fluffy-HAFSs had a significantly higher ALP activity than hMSCs on the HAFMs, which means that a promoted osteogenic differentiation of hMSCs was achieved on fluffy-HAFSs compared to that on HAFMs. Mineralization of hMSCs on different scaffolds was observed using alizarin red S staining. As shown in Fig. 8B and C, after 14 days of culture, hMSCs on fluffy-HAFSs exhibited more obvious positive staining of alizarin red S than hMSCs on HAFMs, which indicated that the fluffy-HAFSs facilitated the mineralization of hMSCs compared to HAFMs.
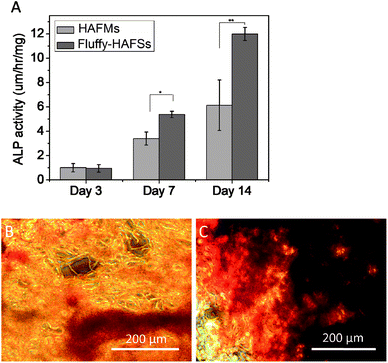 |
| Fig. 8 (A) ALP activity of hMSCs cultured on different scaffolds; Alizarin red S staining of hMSCs cultured on HAFMs (B) and fluffy-HAFSs (C). Data are mean ± SD, n = 4, *p < 0.05, **p < 0.01. | |
In previous studies, Yang and colleagues demonstrated that biodegradable and porous 3-D β-TCP scaffolds could be used to co-culture two cell types and deposit bioactive materials.46–49 Our results suggested that fluffy-HAFSs played a significant role in cell proliferation and differentiation, and which indicated that fluffy-HAFSs can be like 3-D β-TCP scaffolds serving as a source of growth factors, such as VEGF, cytokines, chemokines and other biological signals, providing bioactive cues for cell proliferation, differentiation and vascularization. In addition, we can also develop pre-vascularized HA scaffolds by co-seeding MSCs and MSC-derived endothelial cells and use them to promote the repair of segmental bone defects.
In addition, this study reinforced our understanding of the cell–matrix interaction in HA scaffold-based bone tissue repairing and regeneration. A biomimetic microenvironment provided bioactive cues, regulating the osteogenic behaviors of hMSCs. Therefore, through the in vitro generation of a cell-derived ECM, the cellular function of the scaffold can be improved. On the other hand, our fluffy-HAFSs can be tailored to defects of various shapes and sizes, easily handled and stored in a clinical setting. We believed that the fluffy-HAFSs could be potential candidates as cell-derived fillers for the applications in orthopedics such as facial reconstructive surgery specifically in children and other unsolved problems for the treatments of bone defects in modern medicine.
Conclusions
In the present study, we developed a novel fluffy HA based fibrous scaffold successfully by an improved electrospinning technique combining with a bio-mineralization technique for HA. The fluffy-HAFSs had numerous deep interconnected pores with a diameter of 65 ± 35 μm; the unique fluffy spatial fibrous structure ensured the hMSCs entering into the interior of fluffy-HAFSs and achieved 3D cell culture. The fluffy-HAFSs significantly promoted cell attachment, proliferation, osteogenic differentiation and mineralization of hMSCs.
Acknowledgements
We acknowledge financial support from the National Natural Science Foundation of China (Grant no. 51103181 and 11204033), the project of Science and Technology New Star of Zhu Jiang in Guangzhou city, the project supported by the Natural Science Foundation of Guangdong Province, China (Grant no. S2013040016083), the Fundamental Research Funds for the Central Universities, the Open Research Fund of State Key Laboratory of Bioelectronics, Southeast University, the Research Fund for the Doctoral Program of Higher Education of China (20120092120042), and the Open Fund of The First Affiliated Hospital, Jinan University Guangzhou.
Notes and references
- G. T. Kim, H. Shin and D. W. Lim, Adv. Funct. Mater., 2012, 22, 2446 CrossRef.
- M. C. Phipps, W. C. Clem, J. M. Grunda, G. A. Clines and S. L. Bellis, Biomaterials, 2012, 33, 524 CrossRef CAS PubMed.
- D. H. Kim, H. Lee, Y. K. Lee, J. M. Nam and A. Levchenko, Adv. Mater., 2010, 22, 4551 CrossRef CAS PubMed.
- M. Deng, S. G. Kumbar, L. S. Nair, A. L. Weikel, H. R. Allcock and C. T. Laurencin, Adv. Funct. Mater., 2011, 21, 2641 CrossRef CAS.
- C. Yu, J. Bianco, C. Brown, L. Fuetterer, J. F. Watkins, A. Samani and L. E. Flynn, Biomaterials, 2013, 34, 3290 CrossRef CAS PubMed.
- C. Y. Tay, S. A. Irvine, F. Y. Boey, L. P. Tan and S. Venkatraman, Small, 2011, 7, 1361 CrossRef CAS PubMed.
- B. Bakhshandeh, M. Soleimani, N. Ghaemi and I. Sabani, Acta Pharmacol. Sin., 2011, 32, 626 CrossRef CAS PubMed.
- H. N. Yang, J. S. Park, D. G. Woo, S. Y. Jeon, H. J. Do, H. Y. Lin, J. H. Kim and K. H. Park, Biomaterials, 2011, 32, 5924 CrossRef CAS PubMed.
- B. Rai, J. L. Lin and Z. X. Lim, Biomaterials, 2010, 31, 7960 CrossRef CAS PubMed.
- X. Hu, S. H. Park, E. S. Gil, X. X. Xia, A. S. Weiss and D. L. Kaplan, Biomaterials, 2011, 32, 8979 CrossRef CAS PubMed.
- D. Z. Yang, K. Yu, Y. F. Ai, H. P. Zhen, J. Nie and J. F. Kennedy, Polymer, 2011, 84, 990 CAS.
- A. J. Meinel, K. E. Kubow, E. Klotzsch, M. Carcia-Fuentes, M. L. Smith, V. Voqel, H. P. Merkle and L. Meinel, Biomaterials, 2009, 30, 3058 CrossRef CAS PubMed.
- L. X. Lü, X. F. Zhang, Y. Y. Wang, L. Ortiz, X. Mao, Z. L. Jiang, Z. D. Xiao and N. P. Huang, ACS Appl. Mater. Interfaces, 2013, 5, 319 Search PubMed.
- T. Wang, X. Y. Ji, L. Jin, Z. Q. Feng, J. H. Wu, J. Zheng, H. Y. Wang, Z. W. Xu, L. L. Guo and N. Y. He, ACS Appl. Mater. Interfaces, 2013, 5, 3757 CAS.
- J. A. Zhou, C. X. Xu, G. Wu, X. D. Gao, L. M. Zhang, Z. C. Zhai, Z. W. Zheng, X. F. Chen and Y. J. Wang, Acta Biomater., 2011, 7, 3999 CrossRef CAS PubMed.
- M. J. Dalby, N. Gadeqaard, R. Tare, A. Andar, M. O. Riehle, P. Herzyk, C. D. Wilknson and R. O. Oreffo, Nat. Mater., 2007, 6, 997 CrossRef CAS PubMed.
- L. Jin, Z. Q. Feng, M. L. Zhu, T. Wang, M. K. Leach and Q. Jiang, J. Biomed. Nanotechnol., 2012, 8, 779 CrossRef CAS PubMed.
- Z. Q. Feng, X. H. Chu, N. P. Huang, M. K. Leach, G. Wang, Y. C. Wang, Y. T. Ding and Z. Z. Gu, Biomaterials, 2010, 31, 3604 CrossRef CAS PubMed.
- L. Jin, T. Wang, Z. Q. Feng, M. K. Leach, J. H. Wu, S. J. Mo and Q. Jiang, J. Mater. Chem. B, 2013, 1, 1818 RSC.
- Z. Q. Feng, X. H. Chu, N. P. Huang, M. K. Leach, G. Wang, Y. C. Wang, Y. T. Ding and Z. Z. Gu, Biomaterials, 2010, 31, 3604 CrossRef CAS PubMed.
- Z. Q. Feng, H. J. Lu, M. K. Leach, N. P. Huang, Y. C. Wang, C. J. Liu and Z. Z. Gu, Biomed. Mater., 2010, 5, 065011 CrossRef PubMed.
- L. Jin, Z. Q. Feng, M. L. Zhu, T. Wang, M. K. Leach and Q. Jiang, J. Biomed. Nanotechnol., 2012, 8, 779 CrossRef CAS PubMed.
- R. Murugan and S. Ramakrishna, Tissue Eng., 2006, 12, 435 CrossRef CAS PubMed.
- M. V. Jose, V. K. Thomas, T. Johnson, D. R. Dean and E. Nyairo, Acta Biomater., 2009, 5, 305–315 CrossRef CAS PubMed.
- W. J. Li, C. T. Laurencin, E. J. Caterson, R. S. Tuan and F. K. Ko, J. Biomed. Mater. Res., 2002, 60, 613 CrossRef CAS PubMed.
- C. Li, C. Vepari, H. J. Jin, H. J. Kim and D. Kaplan, Biomaterials, 2006, 27, 3115 CrossRef CAS PubMed.
- S. Z. Fu, P. Y. Ni, B. Y. Wang, B. Y. Chu, J. R. Peng, L. Zheng, X. Zhao, F. Luo, Y. Q. Wei and Z. Y. Qian, Biomaterials, 2012, 33, 8363 CrossRef CAS PubMed.
- F. Y. Zheng, S. G. Wang, S. H. Wen, M. W. Shen, M. F. Zhu and X. Y. Shi, Biomaterials, 2013, 34, 1402 CrossRef CAS PubMed.
- M. E. Frohbergh, A. Katsman, G. P. Botta, P. Lazarovici, C. L. Schauer, U. G. K. Wegst and P. I. Lelkes, Biomaterials, 2012, 33, 9167 CrossRef CAS PubMed.
- M. C. Phipps, W. C. Clem, J. M. Grunda, G. A. Clines and S. L. Bellis, Biomaterials, 2012, 33, 524 CrossRef CAS PubMed.
- S. S. Kim, M. S. Park, O. Jeon, C. Y. Choi and B. S. Kim, Biomaterials, 2006, 27, 1399 CrossRef CAS PubMed.
- X. Xu, X. Chen, A. Liu, Z. Hong and X. Jing, Eur. Polym. J., 2007, 43, 3187 CrossRef CAS PubMed.
- X. Liu, L. A. Smith, J. Hu and P. X. Ma, Biomaterials, 2009, 30, 2252 CrossRef CAS PubMed.
- H. W. Kim, J. H. Song and H. E. Kim, Adv. Funct. Mater., 2005, 15, 1988 CrossRef CAS.
- S. A. Catledge, W. C. Clem, N. Shrikishen, S. Chowdhury, A. V. Stanishevsky, M. Koopman and Y. K. Vohra, Biomed. Mater., 2007, 2, 142 CrossRef CAS PubMed.
- W. G. Cui, X. H. Li, C. Y. Xie, J. G. Chen, J. Zou, S. B. Zhou and J. Weng, Polymer, 2010, 51, 2320 CrossRef CAS PubMed.
- X. H. Liu, L. A. Smith, J. Hu and P. X. Ma, Biomaterials, 2009, 30, 2252 CrossRef CAS PubMed.
- P. Dinarvand, E. Seyedjafari, A. Shafiee, A. B. Jandaghi, A. Doostmohammadi, M. H. Fathi, S. Farhadian and M. Soleimani, ACS Appl. Mater. Interfaces, 2011, 3, 4518 CAS.
- F. Peng, M. T. Shaw, J. R. Olson and M. Wei, J. Phys. Chem. C, 2011, 115, 15743 CAS.
- K. L. Kilpadi, A. A. Sawyer, C. W. Prince, P. L. Chang and S. L. Bellis, J. Biomed. Mater. Res., Part A, 2004, 68, 273 Search PubMed.
- C. F. Brunk, K. C. Jones and T. W. James, Anal. Biochem., 1979, 92, 497 CrossRef CAS.
- G. Wei and P. X. Ma, Biomaterials, 2004, 25, 4749 CrossRef CAS PubMed.
- C. H. He, G. Y. Xiao, X. B. Jin, C. H. Sun and P. T. Ma, Adv. Funct. Mater., 2010, 20, 3568 CrossRef CAS PubMed.
- E. Seyedjafari, M. Soleimani, N. Ghaemi and I. Shabani, Biomacromolecules, 2010, 11, 3118 CrossRef CAS PubMed.
- C. J. Grande, F. G. Torres, C. M. Gomez and M. C. Bañó, Acta Biomater., 2009, 5, 1605 CrossRef CAS PubMed.
- Y. Kang, S. Kim, M. Fahrenholtz, A. Khademhosseini and Y. Yang, Acta Biomater, 2013, 9, 4906 CrossRef CAS PubMed.
- Y. Kang, S. Kim, J. Bishop, A. Khademhosseini and Y. Yang, Biomaterials, 2012, 33, 6998 CrossRef CAS PubMed.
- Y. Kang, A. Scully, D. A. Young, S. Kim, H. Tsao, M. Sen and Y. Yang, Eur. Polym. J., 2011, 47, 1569 CrossRef CAS PubMed.
- Y. Kang, S. Kim, A. Khademhosseini and Y. Yang, Biomaterials, 2011, 32, 6119 CAS.
Footnote |
† Electronic supplementary information (ESI) available: Sectional drawing of the special hemispherical collector, photos of the special collector during the electrospinning process and the fluffy PLLA nanofibrous scaffold, and the method for the measurement of the water absorption rate of different scaffolds. See DOI: 10.1039/c3tb21219j |
|
This journal is © The Royal Society of Chemistry 2014 |
Click here to see how this site uses Cookies. View our privacy policy here.