Acellular biomaterials in mesenchymal stem cell-mediated endogenous tissue regeneration
Received
1st October 2013
, Accepted 28th October 2013
First published on 29th October 2013
Abstract
The functions of acellular biomaterials have evolved from a space filler and mechanical support to biological tissue replacements that guide endogenous tissue regeneration. Delivery of acellular materials avoids high cost of production and scientific and regulation barriers associated with cellular systems. The advent of tissue specific stem cells and advances in biomaterials have created new opportunities for refining the properties and identifying new applications for the acellular materials. In particular, increasing knowledge of mesenchymal stem cell in vivo identity and their roles in endogenous tissue regeneration has provided a target population of resident tissue stem cells and scientific foundation upon which the material properties can be optimized. This article discusses the progress in tissue-specific stem cells, their role in endogenous tissue repair, and methods that direct resident MSC migration and differentiation. These concepts are then discussed in the case of guided tissue regeneration to highlight the application and challenges of acellular biomaterials in clinical applications.
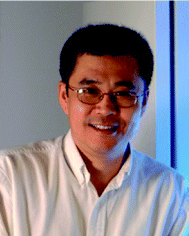 Teng Ma | Teng Ma is a Professor of Chemical and Biomedical Engineering at Florida State University, USA. He received his B.S. and Ph.D. in chemical engineering from Tianjin University, China, and the Ohio State University, Columbus, OH, respectively. Afterward, he was a postdoctoral fellow at the Ohio State University Medical Center. His laboratory is engaged in developing biomimetic scaffolds and bioreactor systems to support tissue regeneration using human mesenchymal stem cells. His research interests are to understand the cellular, physiological, and biomechanical processes of tissue regeneration and to develop enabling technology to guide tissue regeneration. |
Introduction
Traditional synthetic materials such as Dacron® have been used as prostheses that fill a space and function mechanically without cells.1 The limitations of the early cell-free biomaterials soon led to the development and construction of tissue engineered products that enable biological tissue replacement. The emergence of stem cells, including both pluripotent stem cells and adult stem cells, has propelled the development of tissue engineered products as they provide a renewable cell source with capacity to differentiate into virtually all tissues. However, the widespread clinical application of the tissue engineered and cell therapy products using stem cells is likely years away from a clinical reality because of issues such as cell selection and delivery, maintenance of cell viability and phenotype, control of cell fate post-transplantation, and safety and regulatory requirements. Increasing knowledge on tissue resident stem cells and their role in tissue renewal and regeneration has led to renewed interest in endogenous tissue repair by mobilizing tissue resident stem cells. In this context, an acellular approach utilizing cell-free biomaterials to harness innate tissue regeneration capacity by interacting with the resident stem cells has the potential to overcome the scientific and regulatory challenges of the cell-based approach.
Endogenous tissue repair is a part of healing response to tissue loss or injury but has limited capacity in adult mammals. Until recently, adult stem cells or tissue-specific progenitor cells have been primarily studied as a renewable cell source for cell therapy or in vitro regeneration of target tissues. A growing body of evidence, however, suggests that many tissues contain a small population of resident stem cells that contribute to tissue homeostasis and regeneration after injury.2 While the concept of tissue resident stem cells and their role in endogenous tissue repair is not new and has been best illustrated by previous work on hematopoietic stem cells, recent studies suggest that tissue resident stem cell populations are relatively widespread and actively participate in tissue maintenance and repair in many organs over the life span of the animal. Over the last five years, progress has been made in elucidating in vivo biology of tissue resident stem cells, especially their in vivo identity, diversity, and physiological functions in tissue repair and regeneration. For example, studies have shown that regeneration of mammalian digit tips is due to the presence of tissue resident, lineage-restricted progenitor cells rather than the pluripotent stem cell population.3,4 Recent studies also suggest the coexistence of quiescent and active adult stem cells in mammals that cooperatively participate in tissue repair and regeneration,5,6 suggesting a robust mechanism to ensure physiological self-renewal and endogenous tissue regeneration in certain tissues. These advances have provided a scientific foundation for endogenous tissue regeneration by mobilizing endogenous stem cells and motivated the development of novel acellular biomaterials that can effectively harness and augment endogenous tissue regeneration potential. Without the delivery of an exogenous cell population, acellular materials must effectively stimulate and recruit the endogenous progenitor cell pools to participate in tissue regeneration and direct their lineage specification and neo-tissue formation. Thus, understanding the mechanism underlying the interactions between the acellular biomaterials and tissue resident stem cells is crucial for the recruitment of target resident stem cells as well as providing biological and biophysical cues for patterned tissue formation and maturation.
Resident tissue-specific stem cells and endogenous tissue repair
Among various tissue resident stem cells, mesenchymal stem cells (MSCs) have been extensively studied and thought to be broadly distributed. The existence of multipotent bone marrow MSCs (BM-MSC) was proposed in 1970s based on the identification of clonogenic populations within the bone marrow that differentiate into chondrogenic, osteogenic, and adipogenic lineages. Recent studies have focused on identifying the MSC in vivo origin and their physiological role in mediating tissue repair. MSCs are a heterogeneous population and consist of multiple subsets that differ in their relative abundance depending on the developmental stage and tissue origin. Among various MSC populations, BM-MSCs are the most studied and their in vivo characteristics are the best characterized. In the bone marrow, the primary MSCs are predominantly in the arterial perivascular space near the inner face of the cortical bone, forming hematopoietic niche in the arterial perivascular area.7 In contrast, perivascular bone marrow MSCs expressing nestin are closely associated with hematopoietic stem cells (HSCs) and required for HSC maintenance in vivo.8 Although MSCs are commonly defined by in vitro properties, Park et al. recently demonstrated that BM-MSCs in residence serve as progenitor cells for osteoblasts and proliferated and differentiated into osteoblasts in normal tissue turnover. These cells also are responsive to injury and have the ability to home to bone sinusoidal vessels where they engraft.9 To enhance the endogenous bone tissue repair mediated by resident BM-MSCs, a method has been developed to direct tissue-resident MSCs to the bone surface to augment bone formation and increase bone mass.10 A synthetic high-affinity peptide mimetic ligand (LLP2A), a specific ligand for activated α4β1 integrin, is attached to alendronate, a bisphosphonate that has a high affinity for bone. In this hybrid LLP2A–Ale compound, alendronate serves as a bone-seeking component, whereas the LLP2A ligand serves as the target ligand that increases MSC homing to the bone surface. Injection of LLP2A–Ale in mice demonstrated increased bone formation by augmenting endogenous bone formation and by directing the transplanted MSCs to the bone. In addition to rodents, the existence of distinct MSC subsets in the perivascular region and endosteal surfaces has also been demonstrated in the human bone marrow,11 suggesting the clinical prospects to augmenting endogenous tissue repair by enhancing resident stem cell migration.
An increasing body of evidence suggests that MSCs or “MSC-like” cells are broadly distributed and reside in virtually all postnatal organs and tissues owing to their perivascular location. “MSC-like” cells that exhibit in vitro MSC characteristics of colony forming unit-fibroblast (CFU-F) formation, tri-lineage potency, and expression of defining surface markers have been isolated from almost all postnatal tissues and organs, including epicardium and human lung tissue.12–14 The widespread distribution of MSCs in virtually all tissues and organs and their striking similarities to pericytes suggest their broader potential as a target resident stem cell population in endogenous tissue regeneration.15–17 In this effort, understanding how these tissue resident MSCs participate in endogenous repair and regeneration is important for the successful formulation of a therapeutic approach to enhance endogenous tissue regeneration. A case in point is the resident lung MSCs (luMSCs) and their role in lung tissue repair. Global gene expression analysis indicates that luMSCs are a unique stromal subpopulation of pulmonary mesenchyme and differ from lung fibroblasts in terms of production of proinflammatory mediators and reparative growth factors. Resident luMSCs also have high levels of telomerase that enable them to contribute substantially to tissue regeneration. Similar to BM-MSCs, luMSCs regulate native tissue repair and protect lung integrity after injury. However, this function is compromised when endogenous MSCs are lost. To exploit MSCs' reparative and regenerative potential, MSC transplantation has been used for the treatment of chronic obstructive pulmonary disease or to repopulate the decellularized mouse lung scaffold for generating functional lung tissue ex vivo.18,19
Targeting MSC or MSC-like cells in endogenous tissue regeneration
The widespread distribution of tissue resident stem cells such as MSCs provides a target cell population for the development of the acellular biomaterials to promote endogenous tissue regeneration (Fig. 1). Key properties of the acellular materials include the ability to mobilize and recruit endogenous stem cells in sufficient numbers, formation of an instructive microenvironment for cell proliferation and lineage specification, and controlled degradation for tissue maturation. During acute tissue injury, stem cell homing is mediated by chemotactic factors such as stromal cell-derived factor (SDF-1) and monocyte chemotactic protein (MCP)-3, although their expression is quite low and only persists for a short period of time. Because MSCs are known to express receptors to the growth factors and chemokines and robustly respond to these signaling molecules in vitro and in tissue repair, incorporation of chemotactic cytokines in the acellular biomaterials enables site-specific delivery and controlled release with increased stability. For example, implantation of the collagen I scaffold incorporated with SDF-1 induced robust SDF-1 dependent MSC migration and in situ repair of partial-thickness cartilage defects in a rabbit.20 Similarly, incorporation of SDF-1α into polycaprolactone (PCL)/gelatin electrospun membranes induced chemotactic migration of BM-MSCs and yielded a 6-fold increase in the amount of bone formation in rat cranial defects.21 To achieve sustained MSC recruitment, thermal responsive poly(N-isopropylacrylamide) (PNIPAAm)-grafted microcapsules have been developed for controlled and long-term delivery of SDF-1α (>30 days) both in vitro and in vivo.22 The SDF-mediated enhancement of cartilage regeneration has also been demonstrated in a study in which implantation of a scaffold-free hyaline cartilage graft transduced with vectors expressing the SDF-1 transgene in nude mice increased recruitment of endogenous stem cells and a subsequent augmentation of in situ chondrogenesis.23 In addition to SDF-1, implantation of anatomically shaped, cell-free scaffolds infused with collagen gel containing transforming growth factor (TGF)-β3 has been shown to regenerate entire humeral condyles in rabbits after radical resection of the original structures. Incorporation of TGF-β3 into the scaffold plays a major role in directing chondrogenic differentiation as well as enhancing cell migration because the TGF-β3 infusion recruits about 130% more cells than in the absence of TGF-β3.24 These results suggest that the incorporation of chemotactic and growth factors into the acellular materials are an effective strategy to enhanced endogenous tissue regeneration.
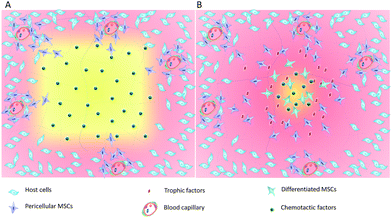 |
| Fig. 1 Schematic of acellular biomaterials in MSC-mediated endogenous tissue regeneration. (A). The acellular biomaterials incorporating chemotactic or growth factors are implanted at the injury site and interact with host cells by releasing the incorporated factors. Gradients of the chemotactic or growth factor induce MSC migration from their pericellular locations, populating the acellular matrix. (B). The implanted acellular biomaterials also provide a structural template for MSC ingrowth, secretion of extracellular matrix proteins, and patterned tissue formation. Additionally, the acellular biomaterials provide binding sites for trophic factors, increasing their stability and function. Ingrowth of MSCs in the acellular biomaterials is accompanied by degradation of the acellular biomaterials and phenotypic differentiation, leading to remodeling of the injury site and functional tissue regeneration. | |
Following recruitment of endogenous progenitor cells, the acellular materials also play important roles in the formation of an instructive microenvironment that sustains cell proliferation and direct lineage specification. In this process, biomaterial properties have been tailored to control local biological, physiological, and biomechanical conditions to enhance tissue regeneration. For example, the cationic chitosan composite membranes have unique ability to locally enrich cell-secreted growth factors and extracellular matrix (ECM) proteins, thereby forming an instructive microenvironment that direct MSC proliferation and osteogenic differentiation.25 In tissue regeneration, physiological conditions such as oxygen tension are known to regulate the in vivo life span of the resident stem cell, from migration, proliferation, to lineage specification. The physical properties of agarose gels have been found to enhance autologous cartilage tissue formation by restricting angiogenesis and promoting hypoxia. Injection of cell-free and growth factor-free agarose gel into subperiosteal space promoted hypoxia-mediated autologous chondrogenesis and ectopic cartilage regeneration26 that are capable of remodeling within a full-thickness osteochondral defection. In a comparative study of cell-laden and cell-free alginate–gelatin hydrogel for the treatment of osteochondral defects, both scaffolds demonstrated regeneration of hyaline-like neo-cartilage and subchondral bone, suggesting the recruitment of endogenous host cells.27 In addition to physiological and biological properties, the biomechanical properties of the acellular biomaterials such as stiffness are known to regulate MSC fate matching the stiffness of the native tissue and guide the tissue development pattern.28 MSCs have been shown to undergo directed migration up stiffness gradients rather than remain stationary, suggesting that both stiffness and stiffness variation regulate MSC behaviors.29 These studies not only demonstrate the potential of the acellular material-mediated endogenous tissue regeneration but also highlight the complexity of the material properties required in endogenous tissue regeneration. Greater understanding of the mechanism of action and delineation of controlling factors in tissue-specific endogenous regeneration continue to play a crucial role in the development of acellular biomaterials.
Guided tissue regeneration – a case study
Barrier membranes used in periodontal tissue regeneration is an early example of acellular materials that have demonstrated clinical efficacy.30 The concept of guided bone regeneration (GBR) has been used in maxillofacial constructive surgery since 1960s.31–34 In the 1980s, guided tissue regeneration (GTR) based on the GBR principle was developed to regenerate the tooth supporting periodontal tissue that includes periodontal ligament (PDL), alveolar bone, and cementum. The initial hypothesis of GTR was that the barrier membranes prevent unwanted growth of surrounding epithelial and fibroblast tissues and provides a secluded space for true periodontal tissue regeneration. Beyond mechanical separation, this process requires migration of undifferentiated progenitors or stem cells from PDL tissue to differentiate into PDL cells and cementoblasts while preventing the invasion of fibroblasts and other soft connective tissue cells. To this end, a membrane barrier needs to selectively guide the migration of “MSC-like” PDL progenitor cells because the surrounding PDL also contains fibroblast cells whose migration could lead to scar tissue formation.35 Hence, the “ideal” barrier membranes not only require appropriate mechanical strength but also biological properties that promote endogenous tissue regeneration, from initial organization of blood clot and bone tissue deposition to lamella bone tissue formation and maturation.
Identification of resident dental stem cells and their role in dental tissue regeneration has benefited the development of GBR/GTR membranes. MSC-like cells have been identified and derived from human pulp tissue, deciduous teeth, and periodontal ligament.36 The dental-derived stem cells appear to be more committed to specific lineages and are responsible for endogenous periodontal tissue regeneration. In GTR, PDL stem cells, a MSC-like cell population, appear to play a major role in periodontal tissue repair by differentiating into PDL-forming cells, mineral-forming cementoblasts, and/or bone-forming osteoblasts. To promote sequential regeneration of hybrid periodontal tissue instead of periodontal repair, the GTR membranes need to function as a scaffold that creates a well-defined extracellular microenvironment where the complex series of events of PDL stem cell recruitment and homing, proliferation, and differentiation are orchestrated. As these complex events are tightly regulated by a multitude of growth factors, incorporation and delivery of chemotactic and growth factors in the GBR/GTR membranes has been an important approach in periodontal tissue regeneration.22,37–39 Studies have also focused on recreation of the complex hierarchical structure of the three periodontal tissues namely cementum, PDL, and alveolar bone. Nanostructured biomaterials in the form of nanofibers or gels closely mimic the natural extracellular matrix network while providing mechanical structure for a greater ability to achieve temporal and spatial control of cellular events. In a recent study, a novel functionally graded membrane consisting of a core-layer and two functional surface-layers was fabricated via multilayering electro-spinning.40 Two functional surface layers composed of a protein/polymer ternary blend (PLCL:PLA:GEL) are designed to interface bone and epithelial tissues, respectively, whereas the core-layer comprised of poly(D,L-lactide-co-caprolactone) (PLCL) is elastic for in vivo placement and mechanical separation (Fig. 2).
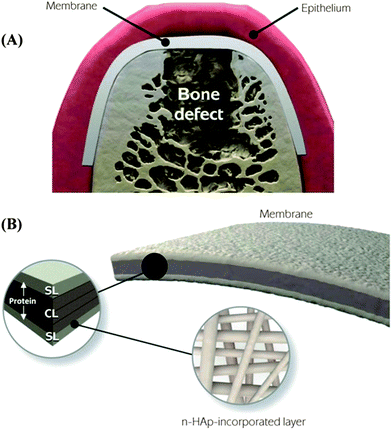 |
| Fig. 2 Schematic illustration of the spatially designed and functionally graded periodontal membrane. (A) Membrane placed in a guided bone regeneration scenario. (B) Details of the core layer (CL) and the functional surface layers (SLs) interfacing bone (n-HAp) and epithelial (MET) tissues. Note the chemical composition step-wise grading from the CL to SLs, i.e., polymer content decreased and protein content increased.40 (Reproduced with permission from ref. 40. Copyright 2011 Elsevier.) | |
Prospects
The identification of MSC-like cells in diverse tissue sources and the increasing understanding of their in vivo biology have provided scientific foundation upon which the properties of the acellular biomaterials can be optimized for endogenous tissue regeneration. The evolvement of GBR/GTR membranes from a mechanical barrier to the delivery vehicle of growth factors and to the scaffolds with graded nanoscale structures and biological properties illustrates how the understanding of endogenous tissues repair has furthered the design and improvement of acellular biomaterials. However, significant challenges remain in the widespread application of acellular materials to induce endogenous tissue repair. First, our knowledge of MSCs and their role in endogenous tissue regeneration is still limited to a few tissue types. This knowledge gap must be bridged because understanding the tissue-specific contribution of the resident MSCs is critical to establish the mechanistic basis for rational designing of acellular materials. Secondly, endogenous tissue regeneration involves complex biological events that cannot be completely recapitulated in vitro. To this end, in vivo studies using advanced imaging and cell tracking techniques can provide invaluable information to decipher the contribution of endogenous stem cells in tissue regeneration. Furthermore, beyond their structural roles, the acellular biomaterials must create a macromolecular milieu of growth factors and ECM proteins that direct the complex biological responses. In this context, appropriate combination of multiple growth factors in the acellular materials and their spatiotemporal coordination will lead to more successful outcomes in tissue regeneration. Finally, the enhancement of endogenous tissue repair by acellular materials is not a replacement of stem cell therapy or transplantation because endogenous regeneration may still not be sufficient in many scenarios or could be further enhanced by the delivery of exogenous cells.41 Nevertheless, harnessing the latent endogenous tissue repair capacity using acellular materials is a promising approach to many devastating diseases.
References
- J. H. Harrison, Am. J. Surg., 1958, 95, 3–15 CrossRef CAS.
- M. Mimeault and S. K. Batra, Stem Cell Rev., 2008, 4, 27–49 CrossRef PubMed.
- Y. Rinkevich, P. Lindau, H. Ueno, M. T. Longaker and I. L. Weissman, Nature, 2011, 476, 409–413 CrossRef CAS PubMed.
- J. A. Lehoczky, B. Robert and C. J. Tabin, Proc. Natl. Acad. Sci. U. S. A., 2011, 108, 20609–20614 CrossRef CAS PubMed.
- L. Li and H. Clevers, Science, 2010, 327, 542–545 CrossRef CAS PubMed.
- N. Barker, S. Bartfeld and H. Clevers, Cell Stem Cell, 2010, 7, 656–670 CrossRef CAS PubMed.
- S. Morikawa, Y. Mabuchi, Y. Kubota, Y. Nagai, K. Niibe, E. Hiratsu, S. Suzuki, C. Miyauchi-Hara, N. Nagoshi, T. Sunabori, S. Shimmura, A. Miyawaki, T. Nakagawa, T. Suda, H. Okano and Y. Matsuzaki, J. Exp. Med., 2009, 206, 2483–2496 CrossRef CAS PubMed.
- S. Mendez-Ferrer, T. V. Michurina, F. Ferraro, A. R. Mazloom, B. D. Macarthur, S. A. Lira, D. T. Scadden, A. Ma'ayan, G. N. Enikolopov and P. S. Frenette, Nature, 2010, 466, 829–834 CrossRef CAS PubMed.
- D. Park, J. A. Spencer, B. I. Koh, T. Kobayashi, J. Fujisaki, T. L. Clemens, C. P. Lin, H. M. Kronenberg and D. T. Scadden, Cell Stem Cell, 2012, 10, 259–272 CrossRef CAS PubMed.
- M. Guan, W. Yao, R. W. Liu, K. S. Lam, J. Nolta, J. J. Jia, B. Panganiban, L. P. Meng, P. Zhou, M. Shahnazari, R. O. Ritchie and N. E. Lane, Nat. Med., 2012, 18, 456 CrossRef CAS PubMed.
- A. Tormin, O. Li, J. C. Brune, S. Walsh, B. Schutz, M. Ehinger, N. Ditzel, M. Kassem and S. Scheding, Blood, 2011, 117, 5067–5077 CrossRef CAS PubMed.
- M. Dominici, K. Le Blanc, I. Mueller, I. Slaper-Cortenbach, F. Marini, D. Krause, R. Deans, A. Keating, D. Prockop and E. Horwitz, Cytotherapy, 2006, 8, 315–317 CrossRef CAS PubMed.
- V. N. Lama, L. Smith, L. Badri, A. Flint, A. C. Andrei, S. Murray, Z. Wang, H. Liao, G. B. Toews, P. H. Krebsbach, M. Peters-Golden, D. J. Pinsky, F. J. Martinez and V. J. Thannickal, J. Clin. Invest., 2007, 117, 989–996 CrossRef CAS PubMed.
- J. J. Chong, V. Chandrakanthan, M. Xaymardan, N. S. Asli, J. Li, I. Ahmed, C. Heffernan, M. K. Menon, C. J. Scarlett, A. Rashidianfar, C. Biben, H. Zoellner, E. K. Colvin, J. E. Pimanda, A. V. Biankin, B. Zhou, W. T. Pu, O. W. Prall and R. P. Harvey, Cell Stem Cell, 2011, 9, 527–540 CrossRef CAS PubMed.
- M. Corselli, C. W. Chen, M. Crisan, L. Lazzari and B. Peault, Arterioscler., Thromb., Vasc. Biol., 2010, 30, 1104–1109 CrossRef CAS PubMed.
- M. Crisan, S. Yap, L. Casteilla, C. W. Chen, M. Corselli, T. S. Park, G. Andriolo, B. Sun, B. Zheng, L. Zhang, C. Norotte, P. N. Teng, J. Traas, R. Schugar, B. M. Deasy, S. Badylak, H. J. Buhring, J. P. Giacobino, L. Lazzari, J. Huard and B. Peault, Cell Stem Cell, 2008, 3, 301–313 CrossRef CAS PubMed.
- M. D. Griffin, S. J. Elliman, E. Cahill, K. English, R. Ceredig and T. Ritter, Stem Cells, 2013, 31, 2033–2041 CrossRef PubMed.
- A. B. Daly, J. M. Wallis, Z. D. Borg, R. W. Bonvillain, B. Deng, B. A. Ballif, D. M. Jaworski, G. B. Allen and D. J. Weiss, Tissue Eng., Part A, 2012, 18, 1–16 CrossRef CAS PubMed.
- B. D'Agostino, N. Sullo, D. Siniscalco, A. De Angelis and F. Rossi, Expert Opin. Biol. Ther., 2010, 10, 681–687 CrossRef CAS PubMed.
- W. Zhang, J. Chen, J. Tao, Y. Jiang, C. Hu, L. Huang, J. Ji and H. W. Ouyang, Biomaterials, 2013, 34, 713–723 CrossRef CAS PubMed.
- W. Ji, F. Yang, J. Ma, M. J. Bouma, O. C. Boerman, Z. Chen, J. J. van den Beucken and J. A. Jansen, Biomaterials, 2013, 34, 735–745 CrossRef CAS PubMed.
- F. M. Chen, H. Lu, L. A. Wu, L. N. Gao, Y. An and J. Zhang, Biomaterials, 2013, 34, 6515–6527 CrossRef CAS PubMed.
- F. Zhang, W. Leong, K. Su, Y. Fang and D. A. Wang, Tissue Eng., Part A, 2013, 19, 1091–1099 CrossRef CAS PubMed.
- C. H. Lee, J. L. Cook, A. Mendelson, E. K. Moioli, H. Yao and J. J. Mao, Lancet, 2010, 376, 440–448 CrossRef CAS.
- K. T. Hunter and T. Ma, J. Biomed. Mater. Res., Part A, 2013, 101, 1016–1025 CrossRef PubMed.
- P. J. Emans, L. W. van Rhijn, T. J. Welting, A. Cremers, N. Wijnands, F. Spaapen, J. W. Voncken and V. P. Shastri, Proc. Natl. Acad. Sci. U. S. A., 2010, 107, 3418–3423 CrossRef CAS PubMed.
- J. C. Schagemann, C. Erggelet, H. W. Chung, A. Lahm, H. Kurz and E. H. Mrosek, Tissue Eng., Part A, 2009, 15, 75–82 CrossRef CAS PubMed.
- A. J. Engler, S. Sen, H. L. Sweeney and D. E. Discher, Cell, 2006, 126, 677–689 CrossRef CAS PubMed.
- J. R. Tse and A. J. Engler, PLoS One, 2011, 6, e15978 CAS.
- P. Cortellini and M. S. Tonetti, Periodontology 2000, 2000, 22, 104–132 CAS.
- P. J. Boyne, J. Dent. Res., 1964, 43, 827 Search PubMed.
- P. J. Boyne, J. Am. Dent. Assoc., 1969, 78, 767 CAS.
- B. Ogiso, F. J. Hughes, A. H. Melcher and C. A. G. Mcculloch, J. Cell. Physiol., 1991, 146, 442–450 CrossRef CAS PubMed.
- C. Dahlin, A. Linde, J. Gottlow and S. Nyman, Plast. Reconstr. Surg., 1988, 81, 672–676 CAS.
- L. Lin, M. Y. Chen, D. Ricucci and P. A. Rosenberg, J. Endod., 2010, 36, 618–625 CrossRef PubMed.
- G. T. Huang, S. Gronthos and S. Shi, J. Dent. Res., 2009, 88, 792–806 CrossRef CAS PubMed.
- S. Elangovan, S. Srinivasan and S. Ayilavarapu, Expert Opin. Biol. Ther., 2009, 9, 399–410 CrossRef CAS PubMed.
- X. E. Dereka, C. E. Markopoulou and I. A. Vrotsos, Growth Factors, 2006, 24, 260–267 CrossRef CAS.
- F. M. Chen, R. M. Shelton, Y. Jin and I. L. Chapple, Med. Res. Rev., 2009, 29, 472–513 CrossRef CAS PubMed.
- M. C. Bottino, V. Thomas and G. M. Janowski, Acta Biomater., 2011, 7, 216–224 CrossRef CAS PubMed.
- L. Shin and D. A. Peterson, Stem Cells Transl. Med., 2013, 2, 33–42 CrossRef CAS PubMed.
|
This journal is © The Royal Society of Chemistry 2014 |
Click here to see how this site uses Cookies. View our privacy policy here.