DOI:
10.1039/C5RA00880H
(Paper)
RSC Adv., 2015,
5, 35693-35703
Superparamagnetic PLGA–iron oxide microspheres as contrast agents for dual-imaging and the enhancement of the effects of high-intensity focused ultrasound ablation on liver tissue
Received
15th January 2015
, Accepted 13th April 2015
First published on 13th April 2015
Abstract
Efforts have been made to develop a multifunctional platform that could provide both diagnostic information and a therapeutic effect. In this study, superparamagnetic poly(lactic-co-glycolic acid) (PLGA)–iron oxide (uSPIO/PLGA) microspheres were prepared for application in ultrasound (US) and magnetic resonance imaging (MRI) and the enhancement of the therapeutic efficiency of high-intensity focused ultrasound (HIFU) on liver tissue through an intravenous injection. Highly dispersed, regular spherical and negatively charged uSPIO/PLGA microspheres were fabricated by a typical double emulsion method in which uSPIO nanoparticles were observed and integrated into PLGA microspheres. The Fe (iron) concentration was determined using atomic absorption spectrometry. In our experiment, the uSPIO/PLGA microspheres showed higher echogenicity than PLGA microspheres and saline in US imaging. Furthermore, the uSPIO/PLGA microspheres appeared to negatively enhance T2-weighted (T2WI) magnetic resonance imaging, with the MR signal intensity decreasing with the increase of the Fe content. The uSPIO/PLGA microspheres were introduced into bovine liver and into rabbits to evaluate the HIFU synergistic ablation efficiency in vitro and in vivo. After the intravascular administration of the uSPIO/PLGA microspheres, the liver tissue was ablated by HIFU, and the tissue exposed to HIFU was subjected to pathological examination to determine its structural variation. Pure PLGA microspheres (without uSPIO) and saline were used as the controls. Our results show that the coagulative necrosis volume and degree of liver tissue in the uSPIO/PLGA group was significantly greater than in the PLGA group and saline group. The employment of the composite uSPIO/PLGA microspheres could significantly enhance dual-modality US/MR imaging and HIFU synergistic therapy with an intravenous administration method.
1. Introduction
Theranostics is a newly emerging concept that involves simultaneous execution of therapeutic and diagnostic approaches for personalized medicine.1 It is well known that ultrasmall superparamagnetic iron oxide (uSPIO) nanoparticles can shorten the T2-weighted transverse relaxation time that decreases the MR signal intensity of targeted tissue compared to the surrounding tissue. Therefore, the contrast between the targeted tissue and the surrounding tissue increases, which could enhance the accuracy of the diagnosis. uSPIO nanoparticles are safe, biocompatible, degradable and can be detected with high sensitivity. Hence, they are a strong candidate for use as an MRI contrast agent.2–4 Multifunctional synthetic biocompatible polymers have been widely studied as efficient carriers for drugs and imaging agents and have also been applied to improve the efficacy of both small molecular-weight therapeutics and imaging agents.5 Poly(lactic-co-glycolic acid) (PLGA) nanoparticles have good biocompatibility and biodegradability and have been widely employed in applications of drugs, tissue engineering and molecular imaging.6–8 Hence, the realization of multifunctional contrast agent fabrication by integrating the advantages of uSPIO nanoparticles and PLGA microspheres would enable multimodal imaging with the combination of two or more imaging modalities or theranostics for simultaneous imaging and therapy.
Many studies have revealed that HIFU (high-intensity focused ultrasound) ablation is a feasible, effective, non-invasive, complication-free treatment that could be used for the clinical destruction of tumor tissue.9–12 However, it faces a series of challenges, including the exponential attenuation of US energy with the increase of depth in tissue, the dissipation of heat energy in high-speed blood flow, and the very small area of tissue destroyed by each exposure, necessitating a long time to ablate a large tumor and thus lowering its therapeutic effects.13–15 Hence, synergistic agents generally have been used to enhance the effects of HIFU ablation. At present, SonoVue is widely employed clinically to improve HIFU ablation effects. A preliminary study confirmed that a self-made Pct-PFCNP (phase-change type perfluorocarbon nanoparticle) emulsion could shorten the HIFU ablation time. The time required for the presence of ablated signals using of Pct-PFCNP (e.g., 120 W cm−2: 4.74 s) is shorter than that using PBS (120 W cm−2: 14.20 s) or lipid control (120 W cm−2: 9.70 s), reducing the required HIFU ablation power and thus enhancing the therapeutic effect of the HIFU ablation of lesions in vivo and in vitro.16 For example, in a previous study, the Pct-PFCNP group exhibited signs of ablation signals when the HIFU acoustic power was 60 W, while the PBS and lipid control groups needed at least 120 W to generate the same signals. On the other hand, superparamagnetic poly(lactic-co-glycolic acid) (PLGA)–iron oxide (uSPIO/PLGA) microcapsules have been developed to enhance the therapeutic efficiency of HIFU breast cancer surgery.17 In this study, the HIFU synergistic effects were observed through intra-tumor injection of synergistic agents.
We aimed to preliminarily verify the HIFU therapeutic efficacy on liver tissue through the intravenous injection of synergistic agents that are concurrently applied for ultrasound (US)/magnetic resonance imaging (MRI) for HIFU guidance. There are several benefits to the combination of MRI and US. MRI is non-invasive imaging technology that presents a high spatial resolution, but it is not real time imaging and is not influenced by the skeleton. US features excellent soft-tissue resolution, high safety and convenience and provides real-time imaging,18,19 but it is easily affected by the skeleton or gas. US and MRI have both been widely accepted for clinical diagnosis.
We prepared a novel multifunctional contrast agent combining uSPIO nanoparticles and PLGA to determine if the uSPIO/PLGA microspheres could offer not only ultrasound or magnetic resonance imaging information for precise diagnosis but also could enhance the efficacy of imaging-guided HIFU therapy.
2. Experimental section
2.1 Preparation of uSPIO/PLGA microspheres
A double emulsion evaporation method was used to synthesize ultraminiature superparamagnetic PLGA–iron oxide microcapsules. First, 100 mg PLGA (50
:
50, MW [molecular weight] = 20
000, Daigang, China) and 200 μL solution of uSPIO (size 10 nm, 10 mg mL−1, Ocean) were added to 5 mL CHCl3. Then, 200 μL double distilled water was poured into the solution after it was completely dissolved. The mixture was emulsified using an ultrasonic oscillator (SONICS & MATERALS Inc., USA) at 130 W for 60 s in an ice-cold environment (approximately 0 °C). Next, 20 mL poly vinyl alcohol (PVA, MV = 25
000, Sigma) solution (5% w/v) was transferred into the above emulsified solution. The secondary emulsion (W/O/W microspheres) was performed using a high-speed dispersion homogenizer at 559 g (FJ300-SH, Shanghai, China) for 5 min. Subsequently, the mixture solution was stirred for 2 h to completely volatilize the CHCl3 at room temperature. The supernatant was discarded after centrifuging (high-speed refrigerated centrifuge, Eppendorf) at 1167 g for 3 min. The precipitate was redissolved and washed with deionized water that was centrifuged a second time. The process was repeated for a total of three times to produce the uSPIO/PLGA microspheres. Finally, the precipitate was diluted to 10 mL and stored at 4 °C for further use. At the same time, pure PLGA microspheres (free of uSPIO) were fabricated for use as a control using the same method. In addition, NaCl solution was acquired for use as the blank control group.
2.2 uSPIO/PLGA microspheres property determination
One drop of uSPIO/PLGA microspheres was pipetted and dispensed onto a copper net that was dried at room temperature. Subsequently, a scanning electron microscope (SEM) (Hitachi, S-3400N; Japan) and a transmission electron microscope (TEM) (Hitachi H-7600, Japan) were employed to observe and characterize the structure of the ultrathin film generated on the copper net. The average diameter and electric potential of the microspheres were detected by a laser particle size analyzer (Zeta SIZER 3000HS, Malvern, USA). Then, a certain amount (2 mL) of DMSO solution was added into the precipitate to dissolve the uSPIO/PLGA microspheres after the first centrifugation. Next, the mixture was centrifuged and washed with deionized water after adding an appropriate amount (2 mL) of HCl. Finally, the solution was diluted with double distilled water, and the Fe content was detected by atomic absorption spectrometry (AAS). In addition, the cell viability of the uSPIO/PLGA microspheres was investigated using the MTT colorimetric procedure. Briefly, after incubation with media containing various concentrations (0, 0.625, 1.25, 2.5, 5.0 and 10 mg mL−1) of uSPIO/PLGA microspheres for 24 h, MDA cell viability testing was performed by the addition of 20 μL of 3-(4,5-dimethylthiazol-2-yl)-2,5-diphenyltetrazolium bromide (MTT, 5 mg mL−1) solution for 4 h. Then, 150 μL DMSO was added to dissolve the formazan crystals. To assess the cell viability, the optical density (OD) was measured at 490 nm with an enzyme-linked immunosorbent assay (ELISA) plate reader. Control experiments were conducted using complete growth culture media without microspheres.
2.3 Ultrasound imaging experiments in a gel
To determine whether the US echo intensity was relative to the Fe concentration, a self-regulating gel mold was made using 3 g agarose gel powder dissolved in 100 mL distilled water. The above mixture was then poured into a container and heated to 100 °C. The columniform capsules were inserted into the gel before the model was cooled down in the 4 °C refrigerator for 2 h. Finally, the self-regulating gel mold was prepared after removing the columniform capsules. The saline, pure PLGA microspheres and uSPIO/PLGA microspheres at concentrations of 0.125, 0.25, 0.5, 1.0, 2.0 mg mL−1 were placed into the gel mold to conduct the in vitro US imaging. The samples were scanned with an ultrasonic diagnostic imaging apparatus (Mylab 90, Esaote, Italy) in conventional B mode using a linear probe that transmits and receives center frequencies of 5.0 and 12.0 MHz. The images of the gel mold were collected at a mechanical index of 0.6, and the focal distance was 40 mm. DFY Image quantitative analysis software (developed by the Institute of Ultrasound Imaging, Chongqing Medical University) was provided to analyze the relationship between the echo intensity and the Fe concentration of the microcapsules. An ellipse sampling frame of the DFY software was selected to be randomly placed in the region of interest of the sample US images. Then, the DFY software automatically measured the echo intensity of the selected region. After repeated measurements, the average echo intensity was obtained using SPSS analysis.
2.4 Animal models
All animals used in the experiments were purchased and bred in the Animal Center of Chongqing Medical University. All animal experiments were approved by our animal ethics committee. In addition, all the experiments and procedures were performed under complete anesthesia. All the animal experiments were conducted under protocols approved by the Institutional Animal Care and Use Committee at Chongqing Medical University.
2.5 In vivo ultrasound imaging
To determine if uSPIO nanoparticles improve the US imaging in vivo, normal New Zealand white rabbits (n = 24) of either gender that were 3–4 months-old and weighed 2.4 ± 0.16 kg were purchased from the Animal Center of Chongqing Medical University. All animals were fasted for 24 h before the experiments, and the abdomen was depilated with 8% Na2S. Then, the rabbits were intravenously anesthetized with 3% pentobarbital solution (1 mL kg−1). US images of the rabbit liver were acquired before and 15 s after the intravenous injection (1 mL kg−1) of the uSPIO/PLGA microspheres, saline and pure PLGA microspheres. The echo intensity of the liver tissue was measured using the same method as described above.
2.6 MRI experiments in a gel
uSPIO/PLGA microspheres, pure PLGA microspheres and the PBS control were applied to confirm the relationship between the uSPIO nanoparticles and MRI imaging and to determine whether the MRI signal intensity was influenced by the concentration of uSPIO nanoparticles. Different concentrations (0, 2.5, 5, 10, 25, and 50 μg Fe per mL) of uSPIO/PLGA microspheres were poured into the self-made gel models. T2-weighted images and the corresponding MRI signal intensities (SI) in the region of interest (ROI) were obtained using a Philips Achieva 3.0T TX MR Scanner (Philips Medical Systems, Netherlands). The imaging parameters were fast field echo (FFE), TR (repetition time) = 80 ms, TE (echo time) = 9 ms, flip = 45 °C, FOV (field of view) = 200 mm, and slice thickness = 3.0 mm. The transverse relaxation rate (1/T2*) was then calculated on the basis of the measured T2* data using MatLab software.
2.7 In vivo MRI
Normal New Zealand white rabbits (n = 24) were randomized into the three groups to conduct the experiment. Saline, pure PLGA microspheres and uSPIO/PLGA microspheres were injected intravenously (1 mL kg−1). Subsequently, T2-weighted images and the SI in the liver tissue were collected before, at 10 s and at 5 min. The following operating parameters were used: FFE, TR = 2500 ms, TE = 80 ms, flip = 90 °C, FOV = 160 mm, and slice thickness = 4.0 mm.
2.8 HIFU ablation experimental equipment
The JC200 HIFU focused ultrasound tumor treatment system (Chongqing HIFU Medical Technology Limited-liability Company) was composed of a therapeutic US unit and a diagnostic US unit under the control of a central processing system. The focal length, diameter and operating frequency of the therapeutic US transducer were 145 mm, 220 mm, and 0.94 MHz, respectively. The focal region was 9.8 mm along the beam axis and 1.3 mm in the transverse direction. Moreover, a 3.5–5 MHz diagnostic US transducer was applied for the real-time imaging to guide the US energy deposition and to assess the coagulation necrosis during the HIFU ablation procedure. The high-energy focused US beam was emitted from the diagnostic transducer to cause coagulative necrosis by transiently raising the temperature of the tissue to >60 °C, while the change in the targeted tissue was real-time monitored by the diagnostic transducer that transmitted a parallel US beam to perform US imaging. Briefly, the main damage mechanism was the thermal effects of the HIFU. The integrated transducer was dipped in degassed water.
2.9 Ex vivo HIFU ablation of bovine liver
Fresh bovine liver specimens, 10 cm × 8 cm × 6 cm, containing few blood vessels and connective tissue, were selected and placed in the saline at 20 °C for 30 min. Then, the degassed and re-warmed bovine liver tissue was immersed in the degassed water. Subsequently, saline, pure PLGA microspheres and uSPIO/PLGA microspheres were vertically injected into the samples. Each bovine liver was injected with nine dots, with three dots in a row. Meanwhile, three rows of the same piece of bovine liver were injected with different contrast agents. In total, five pieces of bovine liver were used to assess the repeatability of the measurements. The diagnostic unit and therapeutic unit were used to monitor and expose the injection areas. In the experiment, the HIFU ablation depth was 15 mm beneath the skin, the exposure duration was 2 s, and the acoustic power was set to 100 W, 120 W, 140 W, 160 W, and 180 W. After HIFU ablation, there was a sharp demarcation between the ablated necrosis and the non-ablated tissue, thus, it was easy to identify the ablated region. The maximum length (L, mm), width (W, mm), and depth (D, mm) of the ablated region where the coagulative necrosis occurred were measured, and the volume (V, mm3) was calculated using the reported formula: V = π × L × W × D/6.20,21
2.10 In vivo HIFU ablation of liver tissue in rabbits
Normal New Zealand white rabbits (n = 24) were randomly assigned to three groups and carefully depilated with 8% Na2S after being anesthetized by an ear vein injection of a 3% pentobarbital solution (1 mL kg−1). Next, the rabbits were fastened to the HIFU treatment bed in the prone position, and degassed water was used to sufficiently cover the liver tissue. The groups received the following treatments: HIFU + saline (I, n = 8), HIFU + PLGA (II, n = 8), and HIFU + uSPIO/PLGA microspheres (III, n = 8). Before the HIFU ablation, the three groups were intravenously injected with saline, pure PLGA microspheres, and uSPIO/PLGA microspheres, respectively (1 mL kg−1). Previous studies revealed that the HIFU ablation acoustic power and exposure duration should be set to 150 W and 5 s, respectively.17,22 The ablation procedure for each plane was started 15 s after the injection.23
During the HIFU treatment, the hyperechoic change of liver tissue was confirmed via real-time imaging. To assess the echo intensity and gray-scale change of the target tissue objectively, DFY image quantitative analysis software (developed by the Institute of Ultrasound Imaging, Chongqing Medical University) was utilized to compare the pre-and post-ablation variation. The measurement method was the same as described above for the US imaging experiments in the gel.
2.11 Examination of tissue
The animals were euthanized with intravenous 3% pentobarbital (1 mL kg−1) and sacrificed immediately in an animal care facility after HIFU ablation. The liver tissue was removed to perform TTC (2,3,5-triphenyltetrazolium chloride) solution staining24 and to measure the coagulative necrosis volume (according to the method and the equation used for the ex vivo HIFU ablation of bovine liver). A 1 cm3 sample was fixed in 4% (W/V) paraformaldehyde for 24 h, embedded in paraffin, and stained with hematoxylin and eosin (HE). The liver tissue, including the ablated zone and the surrounding zone, was sliced into 4 μm sections and subjected to pathological examination using light microscopy (Olympus, BX51, Japan). The samples were sent to the Department of Pathology in Chongqing Medical University, and the staff in that department performed a blind pathological examination. Another 1 mm3 tissue sample was fixed with glutaraldehyde and osmium acid, embedded in epoxy resin, and sliced into ultra-thin sections. Ultrastructural alterations of the targeted tissues were determined using TEM.
2.12 Statistical analysis
All statistical analyses were performed with SPSS 21.0 software (International Business Machines Corporation, New York, USA). Analysis of variance (ANOVA) was used to compare the differences between the groups. The difference was significant if the P value was less than 0.05.
3. Results and discussion
3.1 Characterization of uSPIO/PLGA microspheres
Scheme 1 illustrates the microstructure of the uSPIO/PLGA microspheres and the HIFU ablation principles. uSPIO/PLGA microspheres formed a brown suspension after dilution with double distilled water. The uSPIO/PLGA microspheres were carefully observed by SEM and exhibited a highly dispersed, homogeneously distributed, and spherical morphology (Fig. 1a). The average size and electric potential of the uSPIO/PLGA microspheres were 692.1 ± 110.9 nm and −23.3 ± 5.99 mV, respectively (Fig. 1c and d). There was no significant change between the appearance and particle size after storing for 2 months in a 4 °C refrigerator, indicating good stability. The average size and electrical potential of PLGA microspheres were 686.8 ± 92.94 nm and −22.7 ± 9.41 mV. These results demonstrated that the addition of uSPIO nanoparticles to the polymer shell does not significantly affect the size distributions and electrical potential of uSPIO/PLGA microspheres. As depicted in Fig. 1b, the uSPIO nanoparticles were shown to be present on the shell of the composite microcapsules using a transmission electron microscope. The number of uSPIO nanoparticles encapsulated in the uSPIO/PLGA microspheres measured by atomic absorption method was 1.048 mg mL−1. Cytotoxicity tests were performed to determine whether the internalized PLGA particles affected cell viability. The MTT assay (Fig. 1e) indicated no change in the OD using particle concentrations ranging from 0 (control) to 10 mg mL−1.
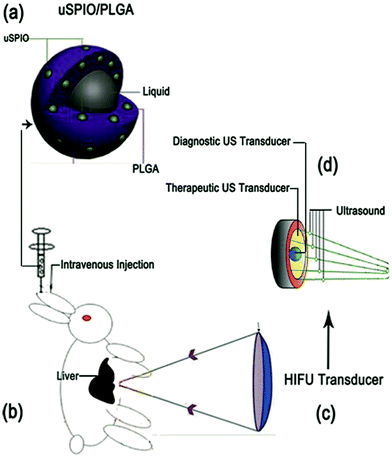 |
| Scheme 1 Schematic illustration of the microstructure of uSPIO/PLGA microspheres and HIFU ablation principles. | |
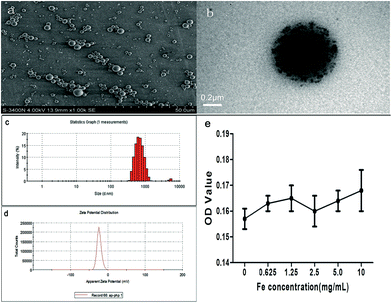 |
| Fig. 1 (a and b) SEM and TEM images of prepared uSPIO/PLGA microspheres, which were highly dispersed and spherical. uSPIO was uniformly present on the shell of microsphere as represented by the black particles. (c and d) Particle size and potential distribution images of uSPIO/PLGA microspheres were detected by laser particle size analysis measurements. (e) The cytotoxicity of uSPIO/PLGA microspheres was evaluated by MTT assay in vitro. The OD value of MDA cells was detected which was treated with different concentrations (0, 0.625, 1.25, 2.5, 5.0, 10 mg mL−1) of uSPIO/PLGA microspheres for 24 h. | |
The introduction of uSPIO nanoparticles endows the microspheres with the property of enhancing T2-weighted MR imaging and US imaging. This property has been shown to be critical for the application of image-guided (US/MR) HIFU ablation. In addition, the introduction of uSPIO nanoparticles demonstrates their other function, namely, serving as synergistic agents to improve HIFU therapeutic efficiency, as investigated in our study. Therefore, the uSPIO/PLGA microspheres were developed for application in US/MR dual-modality imaging and for enhancing the therapeutic efficacy of HIFU ablation in this work. Previously, we successfully introduced these uSPIO nanoparticles into the non-invasive therapeutic field by subcutaneous injection for synergistic US-guided HIFU breast cancer surgery.17 The results demonstrated that the introducing of these particles could efficiently enhance the imaging and HIFU therapeutic efficiency. In this previous work, the uSPIO nanoparticles were subcutaneously injected into rabbits with breast cancer to investigate the HIFU synergistic effect in superficial organs. By contrast, in the present study, the prepared uSPIO/PLGA microspheres were intravenously injected into rabbits through the ear vein to preliminarily investigate the capability of using uSPIO/PLGA microspheres as contrast agents for liver US/MR imaging and for further US focusing to enhance the acoustic energy deposition and improve the therapeutic efficiency.
3.2 Ultrasound imaging experiments in a gel
uSPIO/PLGA microspheres displayed punctiform, tiny, and homogeneous hyperechogenicity compared to saline and pure PLGA microspheres. Fig. 2a indicates that the US echo intensity tended to increase with the concentration of uSPIO/PLGA. In addition, the relationship between the acoustic intensity analyzed by DFY image quantitative analysis software and the Fe concentration (Fig. 2b) also demonstrated this phenomenon. This result may show that the uSPIO nanoparticles integrated in microspheres contributed to US backscattering echo intensity. Some explanations have been put forward for this observation. On one hand, research has revealed certain substances existing in the shell or the multiple shell structure can alter the surface tension of the microbubble thereby causing this type of enhancement of US imaging.25 On the other hand, Yang et al. demonstrated that both the uSPIO nanoparticles in the shell of the microspheres and the polymer shell boosted the acoustic impedance giving rise to stronger scattering signals.26
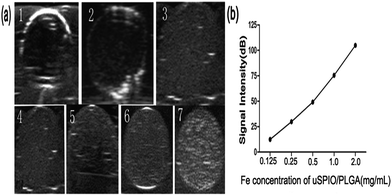 |
| Fig. 2 (a and b) Images of ultrasound imaging experiments in a gel and the corresponding signal intensities of uSPIO/PLGA microspheres at different concentrations (1: saline, 2: pure PLGA microspheres, 3–7: uSPIO/PLGA microspheres at concentrations of 0.125, 0.25, 0.5, 1.0, 2.0 mg mL−1). | |
3.3 Ultrasound imaging in vivo
The US images of the liver samples in three groups (saline, PLGA microspheres and uSPIO/PLGA microspheres) were collected before and at 15 s after intravenous injection. Fig. 3a–c illustrates that the US echo intensity of the whole liver tissue was clearly enhanced in the uSPIO/PLGA microspheres group compared to the pure PLGA microspheres and saline groups (*P < 0.05), in which no significant change was observed (#P > 0.05).
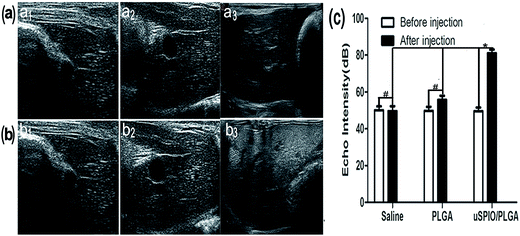 |
| Fig. 3 (a and b) In vivo ultrasound images of liver tissue in rabbits before (a1, a2, a3) and after (15 s: b1, b2, b3) injecting saline, pure PLGA microspheres, and uSPIO/PLGA microspheres, respectively. (c) The corresponding signal intensity of liver tissue after the injection of different agents (#P > 0.05; *P < 0.05). There was no clear echo intensity change of the whole liver tissue in rabbits before and after injecting saline, pure PLGA microspheres (#P > 0.05). The echo intensity of the whole liver tissue in rabbits was significantly enhanced after the injection of the uSPIO/PLGA microspheres compared to the intensity before injection and after injection of saline, pure PLGA microspheres (*P < 0.05). | |
It has been reported that microbubbles consisting of a polymer shell and a specific gas can serve as contrast agents to enhance US imaging because their acoustic impedances apparently differ from those of the surrounding tissue or fluid.27,28 Other studies have revealed that some solid nanoparticles, such as superparamagnetic iron oxide (SPIO) nanoparticles, silica and polystyrene, can boost acoustic impedance, produce stronger backscattered signals, and consequently provide US contrast enhancement.29–31 Due to the existence of uSPIO nanoparticles in the uSPIO/PLGA microspheres, they can change the acoustic environment of the tissue and improve the acoustic impedance and thereby the US echo intensity. There is an increasing trend of echo intensity with increasing concentration of uSPIO/PLGA microspheres. The above mechanisms demonstrate that uSPIO/PLGA microspheres could improve the US echo intensity compared with saline and pure PLGA microspheres in gel experiments and in vivo (Fig. 2 and 3).
Preliminary studies indicated that PLGA acts as the shell of the contrast agent, which is made of amphipathic organic molecular compounds that are biodegradable, nontoxic, and biocompatible for biomedical applications.32 Upon hydrolysis, the PLGA can rapidly disintegrate into metabolizable parts, such as glycolic acid and lactic acid, that are eliminated from the body through the citric acid cycle.33 PLGA is considered an indispensable component in the design of biodegradable multifunctional nanocapsules for biomedical engineering due to its high biosafety.34 Moreover, many studies describe that PLGA is currently widely used to carry drugs and prepare contrast agents.35 As a consequence, PLGA was used as the material of the shell in this research to make the contrast agents more stable.
The above descriptions preliminarily illustrate that uSPIO/PLGA microspheres have the capability to efficiently enhance US imaging in gel experiments and in vivo.
3.4 MR imaging experiments in gel
Samples containing different Fe concentrations were sequentially placed into gel models in the specified order. The darkness of the MR images decreased as the Fe concentration increased. The corresponding MRI signal intensity gradually decreased with the increasing Fe concentration, as displayed in Fig. 4a and b. The relationship between the relaxation rate (1/T2*) and Fe concentration is displayed in Fig. 4c. These results confirm that the uSPIO/PLGA microspheres can serve as effective MRI contrast agents. In light of some previous studies,36,37 ultraminiature superparamagnetic iron oxide (uSPIO) nanoparticles could enable uSPIO/PLGA microspheres to act as magnetic contrast agents for T2-weighted MR imaging, which could negatively enhance the T2-weighted MRI images. This observation indicates that the MR images of the targeted tissue that were injected with uSPIO/PLGA microspheres would become darker compared with the tissue before injection or the surrounding non-targeted tissue. Therefore, this approach may improve the contrast between the targeted tissue and non-targeted tissue, which is beneficial to detect features of interest. This effect occurs through the dipolar reciprocal action of the magnetic moment of the protons in water and the microspheres.
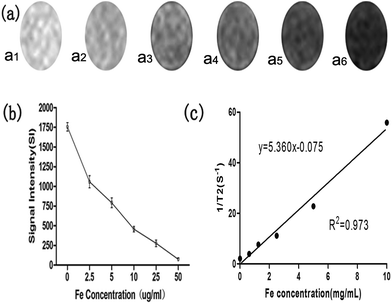 |
| Fig. 4 (a and b) T2-weighted images from MR imaging experiments in gel and corresponding signal intensity of uSPIO/PLGA microspheres with different Fe concentrations (a1: 0, a2: 2.5, a3: 5, a4: 10, a5: 25, a6: 50 μg mL−1) in vitro. (c) A linear relationship was obtained between the transverse relaxation rate (1/T2*) and the Fe concentration (0, 0.625, 1.25, 2.5, 5.0, 10.0 mg mL−1) in the uSPIO/PLGA microspheres. | |
3.5 MR imaging in vivo
To maximize the utilization of the uSPIO/PLGA microspheres as contrast agents for MR imaging, liver tissue from rabbits was employed in the imaging experiment. As indicated in Fig. 5a–c, images were acquired before and at 15 s and 5 min after the intravenous administration of uSPIO/PLGA microspheres, pure PLGA microspheres and saline. There was a significant negative enhancement in the liver tissue in the uSPIO/PLGA microspheres group, while the pure PLGA microspheres and saline did not produce a similar change. The signal intensity of the region-of-interest decreased markedly compared to the previous state and to that of the other two groups (Fig. 5d, #P < 0.05, *P > 0.05). No significant differences were identified between the pure PLGA microsphere group and the saline group in either the enhanced T2-weighted images or the quantitative signal intensity.
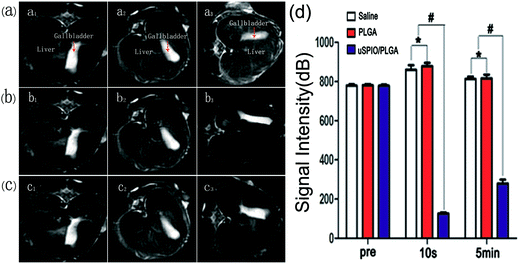 |
| Fig. 5 (a–c) In vivo T2-weighted MRI images and (d) corresponding signal intensity of liver tissue before (a1, a2 and a3) and after (15 s: b1, b2 and b3; 5 min: c1, c2 and c3) the injection of different agents (a1, b1, c1: saline; a2, b2, c2: PLGA; a3, b3, c3: uSPIO/PLGA microspheres) in vivo (*P < 0.05, #P > 0.05). | |
It is significant that uSPIO can produce a predominant T2 relaxation effect, consequently reducing the reduction of the signal of T2-weighted images. This finding can be explained by the heterogeneity of the magnetic field around the nanoparticles, which induces the dephasing of the proton magnetic moments through the diffusion water molecules. Consequently, the T2-weighted transverse relaxation time is shortened.38,39 The MR signal intensity of the uSPIO/PLGA decreased as the concentration of Fe increased, which demonstrates that uSPIO/PLGA microspheres show negatively enhanced imaging as MR contrast agents.
In summary, with the development of medicine and a greater requirement for molecular imaging techniques, multifunctional contrast agents, used not only for various imaging examinations but also to carry genes or medicine for treatment of diseases, have become a research area of interest.40 This type of contrast agent can be applied for multimodal imaging, which is of great significance in reasonably utilizing medical resources, lowering the burden on patients, and enabling imaged-guided therapy in real-time. In our experiment, the prepared uSPIO/PLGA microspheres played an important role in US and MR dual-mode biological imaging and were conducive to the subsequent imaging-guided HIFU ablation therapy.
3.6 Ex vivo HIFU synergistic effects
The achieved uSPIO/PLGA microspheres could potentially overcome the disadvantages of organic microbubbles through their stable nature and excellent biocompatibility, suggesting their capability to enable HIFU synergistic therapy. As shown in Fig. 6, the mean volume of tissue coagulated by HIFU in the group that received uSPIO/PLGA microspheres was greater than the volume of the other two groups that received saline and pure PLGA microspheres (#P < 0.05). There was no significant difference between the saline and pure PLGA microspheres groups (*P > 0.05). Concurrently, the coagulative necrosis area of the bovine liver increased with enhancement of the HIFU acoustic power. This effect is very beneficial for HIFU therapy because high US energy may damage normal tissues in the acoustic propagation paths. The higher necrosis efficiency of the uSPIO/PLGA microspheres might be ascribed to the enhanced ultrasonic absorption in tissues and intensified cavitation. The uSPIO/PLGA microspheres could improve acoustic impedance and change the acoustic environment, which could enhance the absorption and deposition of US energy to increase the temperature of the targeted tissue. Furthermore, the uSPIO nanoparticles may act as extrinsic cavitation nuclei that can lower the cavitation threshold, thereby enhancing the cavitation effect. Based on these findings, it may be promising to utilize uSPIO/PLGA microspheres as HIFU synergistic agents.
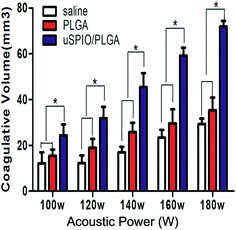 |
| Fig. 6 The coagulative necrosis volume of bovine liver under different HIFU acoustic power (100, 120, 140, 160, and 180 W) at the same exposure duration (2 s) after injecting the different agents of saline, pure PLGA microspheres and uSPIO/PLGA microspheres. | |
3.7 HIFU synergy effects in vivo
In three groups (I: HIFU + saline, II: HIFU + PLGA, III: HIFU + uSPIO/PLGA), the liver tissue was ablated by HIFU under specific ablation parameters (acoustic power 150 W, exposure duration 5 s) after intravenously injecting uSPIO/PLGA microspheres, pure PLGA microspheres and saline, respectively. Immediately, the various gray scale and hyperechoic regions within the focal volume appeared clearly (Fig. 7a and b). The echo signal intensity was significantly higher in group III compared with that in group I and group II after HIFU ablation (Fig. 7c, #P < 0.05). Concurrently, the echo intensity was distinctly enhanced in group II and group III after ablation (Fig. 7c, *P < 0.05). No obvious change was observed in group I before and after HIFU ablation (Fig. 7c, **P > 0.05). Consequently, these findings revealed that uSPIO/PLGA contrast agents could induce the strongest synergistic effect.
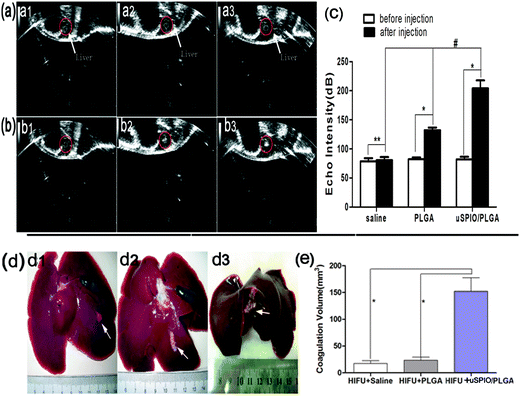 |
| Fig. 7 (a and b) The ultrasound images of the liver tissue in rabbits in the HIFU ablated region before and at 15 s after injecting saline (a1, b1), pure PLGA microspheres (a2, b2) and uSPIO/PLGA microspheres (a3, b3), respectively. (c) The echo intensity of liver tissue in rabbits before and after injecting different agents in the HIFU ablated region (#P < 0.05, *P < 0.05, **P > 0.05). (d) Coagulative necrosis region of liver tissue exposed to HIFU ablation by macroscopic inspections after injecting different agents (d1: saline, d2: pure PLGA microspheres, d3: uSPIO/PLGA microspheres). The necrotic tissue expressed in gray (white arrow), and the non-ablated tissue appeared red. (e) The coagulative volume of different groups (I: HIFU + saline; II: HIFU + PLGA; III: HIFU + uSPIO/PLGA microspheres) after HIFU ablation (*P < 0.05). | |
Next, the liver tissues were removed from the rabbits to calculate the ablation volume. The volume of the liver tissue in which coagulative necrosis occurred in group III was larger than in the control groups (I and II) under the same conditions (Fig. 7d and e). Considering the above findings, uSPIO/PLGA microspheres could serve as synergistic agents that notably increase the ablation efficiency of HIFU at low power.
HIFU has the advantages of minimal trauma, minimal complications, outpatient treatment, fast recovery, and a fast return to normal activities.41,42 The main mechanisms are the thermal effect and the cavitation effect.43 For the thermal effect, a high-energy US beam focuses on a targeted region, localizing the deposition of thermal energy and rapidly raising the tissue temperature (by more than 60 °C). Therefore, this effect may cause protein denaturation and coagulation necrosis. The cavitation effect changes the structure and function of the cell membrane and kills cells using the shock wave and jet flow produced by acoustic cavitation. Several other impacts can also contribute to the HIFU ablation, such as the mechanical effect, acoustic streaming and shear stresses. However, US energy attenuates during transmission through biological tissue, which reduces the US energy deposition and limits the efficiency of the HIFU ablation therapy. Thus, it is necessary to develop a contrast agent to enhance the therapeutic efficiency.
Some studies have indicated that uSPIO is a wave-absorbing material that could be heated in an alternating current magnetic field.44 The presence of uSPIO may have altered the acoustic environment to enhance the absorption of US energy and US energy deposition to increase the temperature of the targeted tissue. The metal uSPIO in the composite microspheres also could improve the heat transfer rate in tissue. The uSPIO nanoparticles are also potential extrinsic cavitation nuclei that can lower the cavitation threshold, enhance the cavitation effect, and improve the heating efficiency of the tissue and the HIFU therapeutic efficiency.45 Previous studies have proven that microbubble agents can improve the effects of HIFU ablation by enhancing the cavitation effect. However, a majority of researchers thought that the cavitation effect ought to be avoided because it is hard to control and might induce an irregularly shaped coagulative volume. Furthermore, the microbubbles are too large to penetrate through the blood vessel endothelium to arrive in the targeted tissue and be easily captured by the reticuloendothelial systems (RES).46–49 Finally, the nano-scale microspheres will produce a concussion under the effect of US waves and cause mechanical damage to tissue. Hence, the multifold mechanisms mentioned above indicate that uSPIO can potentially promote HIFU ablation efficiency ex vivo and in vivo (Fig. 7 and 8). Subsequently, uSPIO/PLGA microspheres were applied in our research to conduct multimodal imaging and assess the synergistic effects for HIFU ablation therapy. The concept was confirmed by the experimental results.
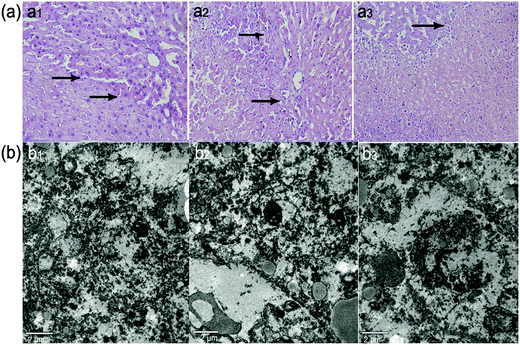 |
| Fig. 8 (a and b) HE staining (a1–a3) and TEM images (b1–b3) of the liver tissue after HIFU ablation. (a1–a3): there exists a significant boundary (black arrow) exists between the ablated and unablated region (400× magnification). (b1–b3): ultrastructural change of the coagulative necrosis region (8000× magnification). A mass of interrupted or undefined cell membranes and nuclear membranes were clearly observed (a1, b1: HIFU + saline; a2, b2: HIFU + PLGA; a3, b3: HIFU + uSPIO/PLGA). | |
3.8 Histological analysis
Microscopic examination revealed destruction to varying degrees within the exposed region. A boundary consisting of invasive white blood cells (reactive zone) lay between the ablated and unablated regions, with a sharp demarcation line. Lysed cell membranes and nuclear fragmentation also were easily observed in the coagulation necrotic tissue (Fig. 8a). Ultrastructural examinations confirmed the irreversible cellular damage in the targeted region, as visualized by TEM. As Fig. 8b illustrates, there was no legible cell structure accompanying the mass of interrupted or undefined cell membranes and nuclear membranes, which suggests the synergistic effect of HIFU ablation caused by uSPIO/PLGA microspheres.
The histological analysis further verifies the synergistic effects of HIFU ablation therapy. HIFU-mediated tissue ablation through mechanical or thermal effects is currently used for the treatment of uterine fibroids and prostate cancers, and these methods are under investigation for breast, pancreas and brain tumors. The local injection of contrast agents in tissues was used in a series of studies on HIFU synergetic ablation instead of intravenous injections,17,50 while the latter is more practical in clinical applications at present. Intravenous administration is less invasive, can be repeated easily if desired, and may be applicable to other tumor types.51 More importantly, studies revealed that microspheres carrying drugs could slow the rate of release of the drug, prolong its circulation time, decrease the drug distribution in the heart and kidney, and enhance the antitumor effect through intravenous injection.52,53 Therefore, intravenous injection was utilized to perform HIFU ablation in our experiment.
Several limitations of our study should be addressed. First, the quantitative research on uSPIO plays a role in enhancing the HIFU ablation but is not explored in our experiment. Second, we did not try to detect or analyze the thermal effects and cavitation effects caused by the uSPIO/PLGA microspheres in the present study. Third, we should extend the present studies to observe the multimodal imaging effects and synergistic effects in other tumor models. Finally, the standardization of therapy response and stability of the manufactured uSPIO/PLGA microspheres under certain biological conditions are not addressed here.
4. Conclusions
Multimodal and multifunctional contrast agents are cutting-edge technologies that maximize the advantages of nanoparticles. uSPIO/PLGA microspheres were successfully prepared for US and MRI dual-modal imaging that are complementary in modern diagnostic practice. Interestingly, from the analysis, we also conclude that uSPIO/PLGA microspheres could act as synergistic agents to improve the therapeutic efficiency of HFIU ablation. Therefore, future research should be dedicated to developing multifunctional and multimodal theranostic agents that can carry chemotherapeutic drugs and genes, which may be critical for future cancer therapies.
Acknowledgements
This work was supported by the National Nature Science of China (Grant no. 81227801, 81130025, 81401423) and Chongqing University Innovation Team Plans (KJTD201303). We are thankful to Qi Wang for his help in HIFU technical support.
Notes and references
- F. Danhier, O. Feron and V. Préat, To exploit the tumor microenvironment: passive and active tumor targeting of nanocarriers for anti-cancer drug delivery, J. Controlled Release, 2010, 148(2), 135–146 CrossRef CAS PubMed.
- J. Wan, W. Cai, X. Meng and E. Liu, Monodisperse water-soluble magnetite nanoparticles prepared by polyol process for high-performance magnetic resonance images, Chem. Commun., 2007,(47), 5004–5006 RSC.
- A. K. Gupta and M. Cupta, Synthesis and surface engineering of iron oxide nanoparticles for biomedical applications, Biomaterials, 2005, 26, 3995–4021 CrossRef CAS PubMed.
- C. Lorenzato, A. Cernicanu, M. E. Meyre, M. Germain, A. Pottier, L. Levy, B. D. de Senneville, C. Bos, C. Moonen and P. Smirnov, MRI contrast variation of thermosensitive magnetoliposomes triggered by focused ultrasound: a tool for image-guided local drug delivery, Contrast Media Mol. Imaging, 2013, 8(2), 185–192 CrossRef CAS PubMed.
- M. P. Melancon and C. Li, Mol. Imaging, 2011, 10(1), 28–42 CAS.
- S. Acharya and S. K. Sahoo, PLGA nanoparticles containing various anticancer agents and tumor delivery by EPR effect, Adv. Drug Delivery Rev., 2011, 63, 170e83 CrossRef PubMed.
- M. A. Wheatley, F. Forsberg, K. Oum, R. Ro and D. El-Sherif, Comparison of in vitro and in vivo acoustic response of a novel 50
:
50 PLGA contrast agent, Ultrasonics, 2006, 44, 360e7 CrossRef PubMed. - J. S. Xu, J. Huang, R. Qin, G. H. Hinkle, S. P. Povoski and E. W. Martin, et al., Synthesizing and binding dual-mode poly (lactic-co-glycolic acid) (PLGA) nanobubbles for cancer targeting and imaging, Biomaterials, 2010, 31, 1716e22 Search PubMed.
- F. Orsi, P. Arnone, W. Chen and L. Zhang, High intensity focused ultrasound ablation: a new therapeutic option for solid tumors, J. Cancer Res. Ther., 2010, 6(4), 414–420 CrossRef PubMed.
- K. Hynynen, O. Pomeroy, D. N. Smith, P. E. Huber, N. J. McDannold and J. Kettenbach, et al., MR imaging-guided focused ultrasound surgery of fibroadenomas in the breast: a feasibility study, Radiology, 2001, 219(1), 176–185 CrossRef CAS PubMed.
- G. Orgera, L. Monfardini, V. P. Della, L. Zhang, G. Bonomo and P. Arnone, et al., High-intensity focused ultrasound (HIFU) in patients with solid malignancies: evaluation of feasibility, local tumour response and clinical results, Radiol. Med., 2011, 116, 734e48 CrossRef PubMed.
- F. Wu, Z. B. Wang, W. Z. Chen, W. Wang, Y. Gui and M. Zhang, et al., Extracorporeal high intensity focused ultrasound ablation in the treatment of 1038 patients with solid carcinomas in China: an overview, Ultrason. Sonochem., 2004, 11, 149e54 Search PubMed.
- E. A. Stewart and W. M. Gedroyc, et al., Focused ultrasound treatment of uterine fibroid tumours: safety and feasibility of a noninvasive thermoablative technique, Am. J. Obstet. Gynecol., 2003, 189(1), 48–54 CrossRef.
- J. E. Kennedy, High-intensity focused ultrasound in the treatment of solid tumours, Nat. Rev. Cancer, 2005, 5(4), 321–327 CrossRef CAS PubMed.
- G. ter Haar, Ultrasound focal beam surgery, Ultrasound Med. Biol., 1995, 21, 1089e100 CrossRef.
- Y. Zhou, Z. Wang, Y. Chen, H. Shen, Z. Luo and A. Li, et al., Microbubbles from Gas-Generating Perfluorohexane Nanoemulsions for Targeted Temperature-Sensitive Ultrasonography and Synergistic HIFU Ablation of Tumors, Adv. Mater., 2013, 25, 4123–4130 CrossRef CAS PubMed.
- Y. Sun, Y. Zheng, H. Ran, Y. Zhou and H. Shen, Superparamagnetic PLGA–iron oxide microcapsules for dual-modality US/MR imaging and high intensity focused US breast cancer ablation, Biomaterials, 2012, 5854–5864 CrossRef CAS PubMed.
- F. Tranquart, N. Grenier, V. Eder and L. Pourcelot, Clinical use of ultrasound tissue harmonic imaging, Ultrasound Med. Biol., 1999, 25(6), 889–894 CrossRef CAS.
- A. Novell, J. M. Escoffre and A. Bouakaz, Ultrasound Contrast Imaging in Cancer, Curr. Mol. Imaging, 2013, 2, 77–88 CrossRef.
- T. Yu, G. Wang, K. Hu, P. Ma, J. Bai and Z. Wang, A microbubble agent improves the therapeutic efficiency of high intensity focused ultrasound: a rabbit kidney study, Urol. Res., 2004, 32(1), 14–19 CrossRef PubMed.
- G. ter Haar, I. Rivens, L. Chen and S. Riddler, High intensity focused ultrasound for treatment of rat tumor, Phys. Med. Biol., 1991, 36(11), 1495–1501 CrossRef CAS.
- Y. Chen, H. Chen, Y. Sun, Y. Zheng, D. Zeng, F. Li, S. Zhang, X. Wang, K. Zhang, M. Ma, Q. He, L. Zhang and J. Shi, Multifunctional Mesoporous Composite Nanocapsules for Highly Efficient MRI-Guided High-Intensity Focused Ultrasound Cancer Surgery, Angew. Chem., Int. Ed. Engl., 2011, 50(52), 12505–12509 CrossRef CAS PubMed.
- D. J. Chunga, S. H. Chob, J. M. Leea and S.-T. Hahna, Effect of microbubble contrast agent during high intensity focused ultrasound ablation on rabbit liver in vivo, Eur. J. Radiol., 2012, 81(4), e519–e523 CrossRef PubMed.
- C. W. Zhou, F. Q. Li, Y. Qin, C. M. Liu, X. L. Zheng and Z. B. Wang, Non-thermal ablation of rabbit liver VX2 tumor by pulsed high intensity focused ultrasound with ultrasound contrast agent: pathological characteristics, World J. Gastroenterol., 2008, 14(43), 6743–6747 CrossRef.
- A. Raisinghani and A. N. DeMaria, Physical principles of microbubble ultrasound contrast agents, Am. J. Cardiol., 2002, 90, 3Je7J CrossRef.
- F. Yang, Y. Li, Z. Chen, Y. Zhang, J. Wu and N. Gu, Superparamagnetic iron oxide nanoparticle-embedded encapsulated microbubbles as dual contrast agents of magnetic resonance and ultrasound imaging, Biomaterials, 2009, 30, 3882e90 Search PubMed.
- E. Quaia, Microbubble ultrasound contrast agent: an update, Eur. J. Radiol., 2007, 17, 1995–2008 CrossRef PubMed.
- D. Cosgrove, Ultrasound contrast agents: an overview, Eur. J. Radiol., 2006, 60, 324–330 CrossRef PubMed.
- S. J. Norton and T. Vo-Dinh, Imaging the distribution of magnetic nanoparticles with ultrasound, IEEE Trans. Med. Imag., 2007, 26, 660e5 Search PubMed.
- F. Scherer, M. Anton, U. Schillinger, J. Henke, C. Bergermann and A. Krüger, et al., Magnetofection: enhancing and targeting gene delivery by magnetic force in vitro and in vivo, Gene Ther., 2002, 9, 102e9 CrossRef PubMed.
- J. Oh, M. D. Feldman, J. Kim, C. Condit, S. Emelianov and T. E. Milner, Detection of magnetic nanoparticles in tissue using magneto-motive ultrasound, Nanotechnology, 2006, 17, 4183e90 CrossRef PubMed.
- M. Stevanovic, I. Bracko, M. Milenkovic, N. Filipovic, J. Nunic and M. Filipic, et al., Multifunctional PLGA particles containing poly(L-glutamic acid)-capped silver nanoparticles and ascorbic acid with simultaneous antioxidative and prolonged antimicrobial activity, Acta Biomater., 2014, 10, 151–162 CrossRef CAS PubMed.
- J. Panyam and V. Labhasetwar, Biodegradable nanoparticles for drug and gene delivery to cells and tissue, Adv. Drug Delivery Rev., 2003, 55, 329–347 CrossRef CAS.
- M. S. Shive and J. M. Anderson, Biodegradation and biocompatibility of PLA and PLGA microspheres, Adv. Drug Delivery Rev., 1997, 28, 5–24 CrossRef.
- Y. Lin, Z. Y. Chen and F. Yang, Ultrasound-based multimodal molecular imaging and functional ultrasound contrast agents, Curr. Pharm. Des., 2013, 19(18), 3342–3351 CrossRef CAS.
- N. Schleich, P. Sibret, P. Danhier, B. Ucakar, S. Laurent, R. N. Muller, C. Jerome, B. Gallez, V. Preat and F. Danhier, Dual anticancer drug/superparamagnetic iron oxide-loaded PLGA-based nanoparticles for cancer therapy and magnetic resonance imaging, Int. J. Pharm., 2013, 447(1–2), 94–101 CrossRef CAS PubMed.
- R. R. Ragheb, D. Kim, A. Bandvopadhyay, H. Chahboune, B. Bulutoglu, H. Ezaldein, J. M. Criscione and T. M. Famy, Induced clustered nanoconfinement of superparamagnetic iron oxide in biodegradable nanoparticles enhances transverse relaxivity for targeted theranostics, Magn. Reson. Med., 2013, 70(6), 1748–1760 CrossRef CAS PubMed.
- J.-M. Lü, X. Wang and C. Marin-Muller, et al., Current advances in research and clinical applications of PLGA based nanotechnology, Expert Rev. Mol. Diagn., 2009, 9(4), 325–341 CrossRef PubMed.
- A. Bjornerud and L. Johansson, The utility of superparamagnetic contrast agents in MRI: theoretical consideration and applications in the cardiovascular system, NMR Biomed., 2004, 17, 465–477 CrossRef PubMed.
- J. J. Li, G. L. Xu, M. F. Gu, G. Y. Luo, Z. Rong and P. H. Wu, et al., Complications of high intensity focused ultrasound in patients with recurrent and metastatic abdominal tumors, World J. Gastroenterol., 2007, 13, 2747e51 Search PubMed.
- F. Danhier, O. Feron and V. Préat, To exploit the tumor microenvironment: passive and active tumor targeting of nanocarriers for anti-cancer drug delivery, J. Controlled Release, 2010, 148, 135–146 CrossRef CAS PubMed.
- D. B. Brown, Concepts, considerations, and concerns on the cutting edge of radiofrequency ablation, J. Vasc. Intervent. Radiol., 2005, 16(5), 597–613 CrossRef PubMed.
- H. Maris and S. Balibar, Negative pressures and cavitation in liquid helium, Phys. Today, 2000, 53, 29–32 CrossRef CAS PubMed.
- CIGNA. Radiofrequency ablation for breast cancer. Cigna healthcare coverage position, 2008, 1–8.
- Y. Kaneko, T. Maruyama and K. Takegami, et al., Use of a microbubble agent to increase the effects of high intensity focused ultrasound on liver tissue, Eur. J. Radiol., 2005, 15, 1415–1420 CrossRef PubMed.
- E. G. Schutt, D. H. Klein, R. M. Mattrey and J. G. Riess, Injectable microbubbles as contrast agents for diagnostic ultrasound imaging: the key role of perfluorochemicals, Angew. Chem., Int. Ed., 2003, 42(28), 3218–3235 CrossRef CAS PubMed.
- B. E. Oeffinger and M. A. Wheatley, Development and characterization of a nano-scale contrast agent, Ultrasonics, 2004, 42(1–9), 343–347 CrossRef CAS PubMed.
- E. Kang, H. S. Min, J. Lee, M. H. Han, H. J. Ahn, I. C. Yoon, K. Choi, K. Kim, K. Park and I. C. Kwon, Nanobubbles from gas-generating polymeric nanoparticles: ultrasound imaging of living subjects, Angew. Chem., Int. Ed., 2010, 49(3), 524–528 CrossRef CAS PubMed.
- Y. Chen, Q. Yin, X. F. Ji, S. J. Zhang, H. R. Chen, Y. Y. Zheng, Y. Sun, H. Y. Qu, Z. Wang, Y. P. Li, X. Wang, K. Zhang, L. L. Zhang and J. L. Shi, Manganese oxide-based multifunctionalized mesoporous silica nanoparticles for pH-responsive MRI, ultrasonography and circumvention of MDR in cancer cells, Biomaterials, 2012, 33(29), 7126 CrossRef CAS PubMed.
- C. Chen, Y. Liu, S. Maruvada, M. Myers and D. Khismatullin, Effect of ethanol injection on cavitation and heating of tissues exposed to high-intensity focused ultrasound, Phys. Med. Biol., 2012, 57(4), 937–961 CrossRef CAS PubMed.
- T. T. Huang, S. Parab, R. Burnett, O. Diago, D. Ostertag, F. M. Hofman, F. L. Espinoza, B. Martin, C. E. lbanez, N. Kasahara, H. E. Gruber, D. Pertschuk, D. J. Jolly and J. M. Robbins, Intravenous administration of retroviral replicating vector, toca 511, demonstrates therapeutic efficacy in orthotopic immune-competent mouse glioma model, Hum. Gene Ther., 2015, 26(2), 82–93 CrossRef CAS PubMed.
- B. L. Wang, Y. M. Shen, Q. W. Zhang, Y. L. Li, M. Luo, Z. Liu, Y. Li, Z. Y. Qian, X. Gao and H. S. Shi, Codelivery of curcumin and doxorubicin by MPEG-PCL results in improved efficacy of systemically administered chemotherapy in mice with lung cancer, Int. J. Nanomed., 2013, 8, 3521–3531 Search PubMed.
- X. Guo, R. Ding, Y. Zhang, L. Ye, X. Liu, C. Chen, Z. Zhang and Y. Zhang, Dual role of photosensitizer and carrier material of fullerene in micelles for chemo-photodynamic therapy of cancer, J. Pharm. Sci., 2014, 103(10), 3225–3234 CrossRef CAS PubMed.
|
This journal is © The Royal Society of Chemistry 2015 |
Click here to see how this site uses Cookies. View our privacy policy here.