DOI:
10.1039/C5RA04028K
(Communication)
RSC Adv., 2015,
5, 32369-32375
New binuclear copper(II) coordination polymer based on mixed pyrazolic and oxalate ligands: structural characterization and mechanical properties†
Received
6th March 2015
, Accepted 23rd March 2015
First published on 23rd March 2015
Abstract
A new inorganic–organic coordination polymer based on a copper(II) binuclear complex coordinated with pyrazole (L1), 1-(hydroxymethyl)pyrazole) (L2) and oxalate (Ox) ligands has been unexpectedly obtained. The crystal structure of this coordination polymer has been unequivocally determined from single crystal X-ray diffraction. One copper(II) center (Cu1) is four-coordinated with two nitrogens (N2, L1 and N3, L2), one oxygen (O1, L1) and one chlorine atom, while the other copper(II) nucleus (Cu2) is five-coordinated with one nitrogen (N1, L1), three oxygens (O1, L2; O2 and O3, Ox) and one chlorine atom, giving slightly distorted square-planar and square-pyramidal geometries, respectively. To the best of our knowledge, such coordination environments have never been previously observed to coexist in the same structure. The terminal chlorine (Cl1) forms the connecting bridge between the planar binuclear [Cu2Cl(Ox)0.5(L1)(L2)]n units ending in an attractive structural framework. An extended layered structure staggered along the b-axis is observed in the supramolecular view. Nanoindentation experiments were carried out and relevant mechanical parameters such as hardness, Young’s modulus, indentation energies and elastic recovery were determined. Additionally, a comparative analysis between the supramolecular structure and the mechanical properties is reported.
1. Introduction
The design of hybrid metal–organic materials (MOMs), which is a burgeoning topic in the crystal engineering domain, is nowadays one of the fastest growing areas in the fields of Materials Science and Supramolecular Chemistry.1,2 This new class of materials attracts significant attention due to their enormous chemical, structural and topological diversity and due to their promising applications, including catalysis, gas storage, magnetism, luminescence, drug delivery, sensing or host/guest inclusion.3–6 Interestingly, such hybrid systems present strong covalent and/or intermolecular bonds to yield 1D chains, 2D layers or 3D frameworks, which incorporate 3d-metal ions and bridging ligands at the same time. The synergy between the organic and inorganic components brings about an outstanding combination of properties that are not common in purely inorganic and organic systems alone. Furthermore, the use of different ligands with similar coordination abilities but different flexibilities as building blocks could be considered as a smart procedure for obtaining periodic frameworks.7–9
In contrast to the large number of recent publications describing the synthesis and characterization of MOM structures, studies focusing on understanding the mechanical properties of these hybrid materials are still scarce due to the lack of proper measurement tools.10 Nonetheless, recent advances in this field are shedding some light on the fundamental structure–mechanical property relationships, which are needed for optimized design and successful performance in diverse technological applications.11 Nanoindentation has emerged as a widely adopted technique for measuring the mechanical properties of different kinds of materials, such as ceramics, organics, inorganics and MOMs at the nanoscale level.12 This technique, where load (P) and penetration depth (h) are measured at high resolution, enables not only the evaluation of the mechanical properties but also the correlation between the measured responses and the underlying structural features and intermolecular interactions.13 This makes nanoindentation a complementary technique to structural analysis for the development of novel smart materials. In general, MOMs have been reported to be mechanically harder than inorganic polymers, while often exhibiting lower density values, similar to those of metallic foams.14
In this paper we present for the first time an inorganic–organic polymeric complex containing two different copper(II) cores with mixed oxalate ion and pyrazolic ligands. This new coordination polymer presents a 2D network along the b-axis formed by layers of stepped chains interconnected by bridging chlorine atoms (Cl1) through the Cu(1)–Cl(1)–Cu(2) bond. The hardness, Young’s modulus, indentation energies and elastic recovery of this Cu(II) supramolecular inorganic/organic framework have been investigated by means of the nanoindentation technique and are compared with similar examples from the literature.
2. Experimental part
2.1 Reagents and synthesis
All reagents were purchased from Sigma-Aldrich and used as received without further purification. 1-(Hydroxymetyl)pyrazole (L2) was synthesized as described previously.15
In order to obtain the copper(II) based coordination complex, pyrazole (L1) and L2 ligands were mixed with CuCl2·2H2O (1
:
1
:
2 molar ratio) for 18 h using ethanol as the solvent under aerobic conditions. The pH was adjusted to 9 with ammonium hydroxide solution (30% NH3). The green powder obtained as the product was washed via vacuum filtration with a few milliliters of cold diethyl ether and dried at room temperature. Minority fraction and translucent blue prism-like monocrystals suitable for X-ray analysis were obtained through the crystallization of the synthesis product using an ethanol–dichloromethane (1
:
1) diffusion technique.
2.2 Characterization techniques
For X-ray crystallographic analysis, a translucent blue prism-like specimen of C8H8ClCu2N4O3 (Mw = 370.71 g mol−1), 0.145 mm × 0.252 mm × 0.290 mm, was used. X-ray intensity data were measured on a D8 Venture system equipped with a multilayer monochromator and a Mo microfocus (λ = 0.71073 Å). A total of 682 frames were collected. The total exposure time was 1.89 hours. The frames were integrated with the Bruker SAINT Software Package using a narrow-frame algorithm. The integration of the data using a monoclinic unit cell yielded a total of 13
166 reflections, to a maximum θ angle of 26.37° (0.80 Å resolution), out of which 2283 were independent (average redundancy 5767, completeness = 99.8%, Rint = 3.44%, Rsig = 2.24%) and 2121 (92.90%) were greater than 2σ(F2). Elemental analysis (C, H, N) of the monocrystal-like specimen was carried out on a Carlo Erba CHNS EA-1108 instrument, separated by a chromatographic column and thermoconductivity detector. Copper elemental analysis was carried out using inductively coupled plasma mass spectrometry (ICPMS) with an Agilent 7500ce model system. Infrared (IR) spectra were recorded on a Perkin-Elmer FT spectrophotometer series 2000, in cm−1 and as KBr pellets. Conductivity measurements were performed at room temperature in 10−3 M methanol solutions, employing a CyberScan CON 500 (Eutech instrument) conductimeter. Electronic spectra were collected on a Kontron-Uvikon 860 in methanol, between 750 and 350 nm.
2.3 Mechanical properties
The mechanical properties (hardness, reduced elastic modulus and elastic recovery) were evaluated by means of nanoindentation, using a UMIS device from Fischer-Cripps Laboratories, equipped with a Berkovich pyramidal-shaped diamond tip, operating in the load control mode. The nanoindentation tests were performed perpendicular to the planar base (100) of the crystal sheet. Other orientations were not possible due to the crystal exfoliation of the material. Indentations using maximum applied load values ranging from 1 to 5 mN were applied to ensure that the maximum penetration depth was much smaller than the crystal thickness. The indentations were separated from each other by 20 μm, to ensure that the stress fields underneath the indents did not overlap with each other. The thermal drift during nanoindentation was lower than 0.05 nm s−1. Proper corrections for the contact area (calibrated with a fused quartz specimen), instrument compliance, and initial penetration depth (a pre-contact load of 0.02 mN was used) were applied. The hardness (H) and reduced elastic modulus (Er) values were derived from the load–displacement curves using the method of Oliver and Pharr.16 From the initial unloading slope, the contact stiffness, S, was determined as: |
 | (1) |
The reduced Young’s modulus was evaluated based on its relationship with the contact area, A, and S:
|
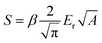 | (2) |
where
β is the King’s factor, which depends on the geometry of the indenter (
β = 1.034 for a Berkovich indenter).
17 The reduced Young’s modulus takes into account the elastic displacements that occur in both the specimen, with Young’s modulus
E and Poisson’s ratio
ν, and the diamond indenter, with elastic constants
Ei and
νi (for diamond,
Ei = 1140 GPa and
νi = 0.07):
|
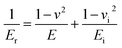 | (3) |
The hardness was calculated from the following expression:
|
 | (4) |
where
Pmax is the maximum load applied during nanoindentation. Finally, the elastic recovery was evaluated as the ratio between the elastic and the total (plastic + elastic) energies during nanoindentation,
Wel/
Wtot. The values of
Wel were calculated as the area between the unloading curve and the displacement axis. In turn,
Wtot is the area between the loading curve and the displacement axis.
18 The results presented in this work are the statistical average of a set of 10 indentations at the applied maximum load.
3. Results and discussion
3.1 Crystal structure of the binuclear copper(II) based coordination polymer
The reaction of L1 and L2 ligands with CuCl2·2H2O (1
:
1
:
2 molar ratio) was carried out in ethanol. The recrystallization of the reaction product afforded minority fraction blue prism-like monocrystals. The X-ray diffraction measurements performed on the obtained monocrystals revealed that the complex crystallizes in the P2(1)/c space group with two independent Cu(II) metal centers having square-planar and square-pyramidal geometries, respectively (Fig. 1). The complex presents a polymeric structure showing a one-dimensional (1D) infinite neutral chain of planar binuclear [Cu2Cl(Ox)0.5(L1)(L2)]n units, wherein both L1–L2 ligand bridges are adjacent the different copper(II) centers. Additional characterization of these monocrystals was carried out.19
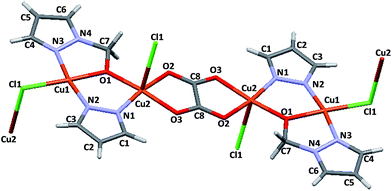 |
| Fig. 1 X-Ray structure of the [Cu4Cl2(Ox)(L1)2(L2)2] complex, where [Cu2Cl(Ox)0.5(L1)(L2)] is the basic monomeric unit of this polymeric chain. Colour scheme: copper, orange; oxygen, red; chlorine, green; nitrogen, blue and carbon, grey. | |
The local coordination environment around the four-coordinated Cu(II) atom (Cu1) is formed by two pyrazolyl (pz) nitrogen atoms (N2 and N3 in L1 and L2 ligands, respectively), one alcohol oxygen atom (O1, L2) and one bridged chlorine atom (Cl1). The coordination sphere of Cu1 can be described as a slightly distorted square-planar geometry with N2–Cu1–O1 and N3–Cu1–Cl1 angles between 81.17(7)° and 94.26(5)°, respectively, which is within the expected range for copper(II) compounds with a square-planar geometry. The Cu1–O1, Cu1–N2 and Cu1–N3 bond distances have similar values in the 1.937–1.945 Å range, indicating a stronger coordination than the Cu1–Cl1 bond (2.25 Å). The [CuClO(Npz)2] core has been previously reported in the literature20–23 but never as a building block of a polymeric structure. Selected values of bond lengths and bond angles for the complex as well as the corresponding crystallographic data are shown in Table 1 and Table 2, respectively.
Table 1 Selected bond lengths (Å) and bond angles (°) for the (a) square-planar and (b) square-pyramidal copper(II) geometries of the complex. Symmetry code: #1 −x + 1,−y + 1,−z
Geometries |
Bond lengths (Å) and bond angles (°) |
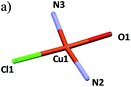 |
Cu(1)–N(2) |
1.9371(17) |
Cu(1)–N(3) |
1.9441(18) |
Cu(1)–O(1) |
1.9448(14) |
Cu(1)–Cl(1) |
2.2569(5) |
N(2)–Cu(1)–O(1) |
89.18(7) |
N(3)–Cu(1)–O(1) |
81.17(7) |
N(2)–Cu(1)–Cl(1) |
95.45(5) |
N(3)–Cu(1)–Cl(1) |
94.26(5) |
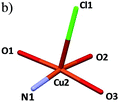 |
Cu(2)–N(1) |
1.9257(17) |
Cu(2)–O(1) |
1.9318(14) |
Cu(2)–O(2) |
1.9679(14) |
Cu(2)–O(3)#1 |
1.9991(14) |
Cu(2)–Cl(1) |
2.795(4) |
N(1)–Cu(2)–O(1) |
89.00(7) |
O(2)–Cu(2)–O(1) |
95.59(6) |
N(1)–Cu(2)–O(3)#1 |
89.92(7) |
O(2)–Cu(2)–O(3)#1 |
84.78(6) |
O(3)#1–Cu(2)–Cl(1) |
92.57(5) |
Table 2 Crystallographic data for the copper(II) complex
Molecular formula |
C8H8ClCu2N4O3 |
Formula weight |
370.71 |
Temperature (K) |
100 (2) |
Wavelength (Å) |
0.71073 |
System, space group |
Monoclinic, P21/c |
![[thin space (1/6-em)]](https://www.rsc.org/images/entities/char_2009.gif) |
Unit cell dimensions |
a (Å) |
10.5078(7) |
b (Å) |
9.1266(6) |
c (Å) |
11.7875(8) |
α (°) |
90 |
β (°) |
99.869(3) |
Γ (°) |
90 |
U (Å3) |
1113.70(13) |
Z |
4 |
Dcalc (mg m−3) |
2.211 |
μ (mm−1) |
4.062 |
F (000) |
732 |
Crystal size (mm3) |
0.290 × 0.252 × 0.145 |
hkl ranges |
−13 ≤ h ≤ 13 |
−11 ≤ k ≤ 11 |
−14 ≤ l ≤ 14 |
2θ range (°) |
2.84 to 26.37 |
Reflections collected/unique/[Rint] |
13166/2283 [R(int) = 0.0344] |
Completeness to θ |
99.8% (θ = 25.242°) |
Absorption correction |
Semi-empirical from equivalents |
Data/restraints/parameters |
2283/54/163 |
Goodness-of-fit on F2 |
1.058 |
Final R indices [I > 2σ(I)] |
R1 = 0.0208, wR2 = 0.0545 |
R indices (all data) |
R1 = 0.0232, wR2 = 0.0559 |
Largest diff. peak and hole (e Å−3) |
0.444 and −0.412 |
Regarding the five-coordinated Cu2 atom, the basal plane is formed by one pyrazolyl nitrogen atom (N1, L1), one alcohol oxygen atom (O1, L2) and two chelated oxygen atoms from the oxalate anion (O2 and O3, Ox). These four coordinated atoms together with the Cu2 ion define an almost perfect planar arrangement. The Cu2 atom is slightly displaced 0.124(2) Å from the basal NO3 plane. The chlorine atom (Cl1) is coordinated to the apical position of the Cu2 site with a longer bond distance of 2.795(3) Å. The coordination sphere of Cu2 can be described as a slightly distorted square-pyramidal geometry with O(2)–Cu(2)–O(1) and O(2)–Cu(2)–O(3) angles between 84.78(6) and 95.59(6)°, respectively, and Cu(2)–O(1), Cu(2)–O(2), Cu(2)–O(3) and Cu(2)–N(1) bond distances of 1.932, 1.968, 1.999 and 1.926 Å, respectively. Such angles and bond distances are within the expected range for copper(II) compounds with square-pyramidal geometry. In the crystal structure of the Cu2 metal centers with square-pyramidal geometry are connected to each other via one oxalate anion (Ox) ligand through a bis-bidentate chelating mode, with adjacent Cu(II) atoms at 5.164 Å (μ-oxalato-1ƙ2O1,O2:2ƙO1a,O2a). The Cu–Ox distances fall within the typical range for oxalate bridged Cu(II) complexes.24–26 The Cu1⋯Cu2 distance of 3.350 Å is out of the limit of the interactive range between the different copper atoms (van der Waals radius of Cu(II) is 2.8 Å). The pyrazole rings are nearly parallel with a dihedral angle of 3.15(1)° between their planes.
The unexpected formation of the oxalate anion in the reaction medium could be attributed to the ethanol oxidation by dissolved O2, favored by the basicity of the medium and the catalytic properties of the copper(II) ions (the reaction took place under aerobic and basic conditions). Copper(II) ions could cause the catalytic activation of O2 and OH− to produce reactive species (i.e. hydroxyl radicals, OH˙−) capable of oxidizing a minor fraction of the ethanol solvent. Using the spectrophotometric method, traces of oxalic acid were found after stirring a basic solution of ethanol and CuCl2 under aerobic conditions.27 Similar oxalate occurrence was reported by Jing Su et al.28 and Mansour Arab et al.29 The possible reactions involved in the synthesis process of the complex presented here are illustrated in Scheme 1.
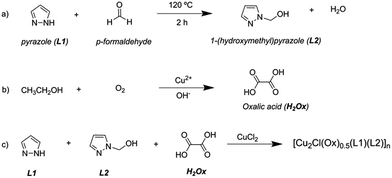 |
| Scheme 1 Chemical reactions involved in obtaining the polymeric complex: synthesis of the L2 ligand (a), in situ formation of oxalic acid (b) and complex formation (c). | |
The “in situ” formation of oxalic acid from solvent during the complex formation reaction has been previously reported.30,31 In addition, there are also previous reports of aerobic alcohol oxidation during the formation of copper coordination complexes or in the presence of copper salts.32–34 In all these cases the alcohol oxidation is related to the catalytic properties of copper(II) ions.
Copper, with a dynamic Jahn–Teller distortion,35 efficiently catalyzes the oxidation of organic compounds under mild conditions and can provide open Lewis acid sites which are effective for catalysing various organic reactions, such as azide–alkyne cycloaddition, oxidative coupling reactions, Henry or Diels–Alder reactions,36–38 or water oxidation,39 among others.
This binuclear copper(II) based coordination polymer owes its particularity to the fact that it is composed of three different ligands, where neither of the [CuClO3Npz] and [Npz–Cu–Ox–Cu–Npz] coordination environments have been reported before.40 Only the [CuClO(Npz)2] core presents a similar coordination sphere to that already described.20–23, Such special coordination spheres provide interesting structures in the network of this complex.
In the supramolecular crystal arrangement, each [Cu4Cl2(Ox) (L1)2(L2)2] molecule is surrounded by 4 other neighbouring molecules in the unit cell. The disposition of these molecules in the unit cell is a typical ABAB structure. Zig-zag layers of polymeric chains interconnected by chloride bridges between the intermolecular copper centers afford a 2D structure along the b axis in the supramolecular framework (Fig. 2A). The chlorine atoms (Cl1) are the bridges between one molecule and the other through the Cu2–Cl1–Cu1 bond with a 93.02° angle. In addition, the intramolecular contacts between the N3⋯Cl1⋯N2 atoms (3.086 and 3.111 Å, respectively) contribute to support the supramolecular network (Fig. 2B). The staggered layered structure determines the mechanical properties measured for this polymer using the nanoindentation technique.
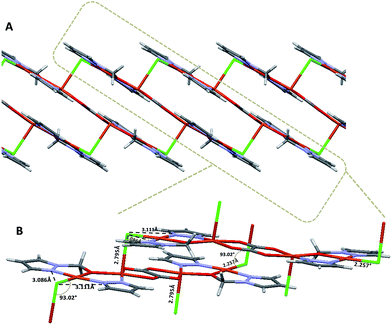 |
| Fig. 2 (A) Polymeric layer structure with zig-zag distribution along the b axis. (B) The close-up view displays the distances and the angles formed by the Cu1–Cl1–Cu2 intermolecular bridges. | |
3.2 Mechanical properties of the binuclear copper(II) based coordination polymer
Representative nanoindentation curves, performed perpendicular to the planar base of the crystal, that corresponds to the (100) face, and up to maximum loads of 1.2 and 2.8 mN, are shown in Fig. 3a and S1.† Measurement of the mechanical properties by nanoindentacion along the other directions was not possible because of the high tendency for the crystals towards brittle fracture. Discontinuities or pop-in events in the loading segments are often observed and are ascribed to the breakage of molecular stacks and the concomitant sudden penetration of the indenter deeper into the sample.
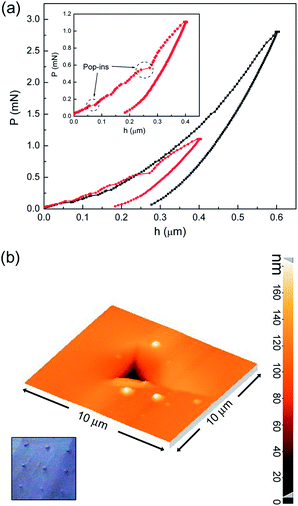 |
| Fig. 3 (a) Representative nanoindentation curves performed perpendicular to the crystal sheet, and up to maximum loads of 1.2 mN (red line) and 2.8 mN (black line). (b) Atomic force microscopy and optical (inset) images of an indentation performed using a maximum load of 2.8 mN. | |
The atomic force microscopy image (Fig. 3b) of an indentation performed using a maximum load of 2.8 mN shows that pile-up effects are virtually absent, while crack formation from the edge of the triangular imprint sometimes occurs, an observation which is in agreement with the occurrence of serrations in the loading segments of the indentation curves (Fig. 3a). The lack of pile-up avoids artifacts related to the determination of the contact area from the contact depth, when using the method of Oliver and Pharr.16
The values of hardness, reduced Young’s modulus, indentation energies and elastic recovery (Wel/Wtot) are shown in Fig. 4a and b. Hardness remains rather constant (H ≈ 0.4–0.5 GPa) as a function of the applied maximum load (Fig. 4a). This value is a bit higher than the ones reported by C. M. Reddy et al.41 for 2-(methylthio)nicotinic acid and similar to values reported by J. C. Tan et al. for different low-symmetry polymorphs of copper phosphonoacetates [Cu1.5(H2O)(O3PCH2CO2)].42 Conversely, the Young’s modulus is a bit lower than the values reported for some other molecular crystals13,42,43 but similar to the values reported by A. Samanta et al. for [OZn4(BDC)3]n, where BDC stands for 1,4-benzenedicarboxylate anion.44 The increase of Er with the applied load indicates a higher resistance against elastic deformation as the penetration depth is progressively increased. This could be due to the larger amount of molecular bonds which hinder the elastic deformation as the applied load is progressively increased. Nevertheless, no clear changes in the elastic energy or the elastic recovery are observed in the range of applied loads used in this study (Fig. 4b). The value of elastic recovery (Wel/Wtot ≈ 0.6) is clearly larger than those of other types of organic–inorganic materials45 and is comparable to the values in some recent reports on 3D inorganic composite films.46
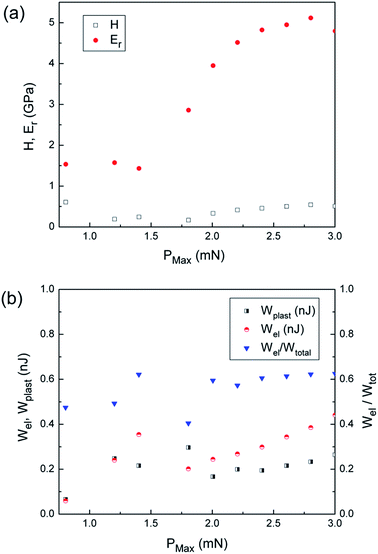 |
| Fig. 4 Values of (a) hardness and reduced Young’s modulus and (b) indentation energies and elastic recovery (Wel/Wtot) of the complex. | |
4. Conclusions
A new binuclear copper(II) complex with a polymeric structure has been unexpectedly obtained from a mixture of pyrazolic ligands and CuCl2 in ethanol. The two copper(II) centers have different coordination numbers and geometries. The Cu1 atom is four-coordinated in the [CuClO(Npz)2] core with a square-planar molecular geometry, while the Cu2 nucleus is five-coordinated with [CuClO3Npz] core and exhibits square-pyramidal geometry. Furthermore, the Cu2 centers of different molecular units are interconnected in the polymeric structure via one unexpected oxalate anion. The terminal chlorine atoms act as bridges between the monomeric units in this coordination polymer. As far as we know, this is the first time that the [CuClO3Npz] and [Npz–Cu–Ox–Cu–Npz] coordination environments are reported. In the supramolecular network an interesting 2D structure with layered architecture formed from polymeric chains interconnected by Cu2–Cl1–Cu1 bridges is formed along the b-axis. Additionally, the values of hardness, Young’s modulus, and elastic recovery of this structure have been determined by means of nanoindentation. The mechanical characterization of this kind of hybrid material is an important asset to crystal engineering since durability and reliability, which are dictated by the mechanical properties, are important factors for their potential applications in sensors, catalysts and gas storage, among others.
Acknowledgements
The financial support from the MAT2011-27380-C02-01 and the MAT2011-27225 research projects from the Spanish MINECO, and the 2014-SGR-1015 and the 2014-SGR-260 projects from the Generalitat de Catalunya are acknowledged. M. D. B. was partially supported by an ICREA-Academia award. A. L_M. thanks the FI-2010 scholarship given by the Government of Catalonia. M. G. acknowledges the support of the Secretary for Universities and Research of the Government of Catalonia and the COFUND Programme of the Marie Curie Actions of the 7th R & D Framework Programme of the European Union for the ‘Beatriu de Pinos’ contract (2013 BP-B 00077). E. P. acknowledges the Spanish MINECO for the ‘Ramon y Cajal’ contract (RYC-2012-10839).
References
- T. R. Cook, Y.-R. Zheng and P. J. Stang, Chem. Rev., 2013, 113, 734–777 CrossRef CAS PubMed.
- M. Eddaoudi, J. Kim, N. Rosi, D. Vodak, J. Wachter, M. O’Keeffe and O. M. Yaghi, Science, 2002, 295, 469–472 CrossRef CAS PubMed.
- I.-H. Park, A. Chanthapally, Z. Zhang, S. S. Lee, M. J. Zaworotko and J. J. Vittal, Angew. Chem., Int. Ed., 2014, 53, 414–419 CrossRef CAS PubMed.
- S. Xiang, Y. He, Z. Zhang, H. Wu, W. Zhou, R. Krishna and B. Chen, Nat. Commun, 2012, 3, 954–963 CrossRef PubMed.
- X.-L. Yang, M.-H. Xie, C. Zou, Y. He, B. Chen, M. O’Keeffe and C.-D. Wu, J. Am. Chem. Soc., 2012, 134, 10638–10645 CrossRef CAS PubMed.
- Y. Zhao, D.-S. Deng, L.-F. Ma, B.-M. Ji and L.-Y. Wang, Chem. Commun., 2013, 49, 10299–10301 RSC.
- L. Croitor, E. B. Coropceanu, D. Chisca, S. G. Baca, J. van Leusen, P. Kögerler, P. Bourosh, V. C. Kravtsov, D. Grabco, C. Pyrtsac and M. S. Fonari, Cryst. Growth Des., 2014, 14, 3015–3025 CAS.
- L. J. McCormick, S. G. Duyker, A. W. Thornton, C. S. Hawes, M. R. Hill, V. K. Peterson, S. R. Batten and D. R. Turner, Chem. Mater., 2014, 26, 4640–4646 CrossRef CAS.
- F. Gul-E-Noor, M. Mendt, D. Michel, A. Pöppl, H. Krautscheid, J. Haase and M. Bertmer, J. Phys. Chem. C, 2013, 117, 7703–7712 CAS.
- J. C. Tan and A. K. Cheetham, Chem. Soc. Rev., 2011, 40, 1059–1080 RSC.
- W. Li, M. S. R. N. Kiran, J. L. Manson, J. A. Schlueter, A. Thirumurugan, U. Ramamurty and A. K. Cheetham, Chem. Commun., 2013, 49, 4471–4473 RSC.
- S. Varughese, M. S. R. N. Kiran, U. Ramamurty and G. R. Desiraju, Angew. Chem., Int. Ed., 2013, 52, 2701–2712 CrossRef CAS PubMed.
- J. C. Tan, J. D. Furman and A. K. Cheetham, J. Am. Chem. Soc., 2009, 131, 14252–14254 CrossRef CAS PubMed.
- S. S. Han and W. A. Goddard, J. Phys. Chem. C, 2007, 111, 15185–15191 CAS.
- W. L. Driessen, Recl. Trav. Chim. Pays-Bas, 1982, 101, 441–443 CrossRef CAS.
- W. C. Oliver and G. M. Pharr, J. Mater. Res., 1992, 7, 1564–1583 CrossRef CAS.
- A. Fischer-Cripps, Nanoindentation, Springer, New York, USA, 2004 Search PubMed.
- E. Pellicer, A. Varea, S. Pané, B. J. Nelson, E. Menéndez, M. Estrader, S. Suriñach, M. D. Baró, J. Nogués and J. Sort, Adv. Funct. Mater., 2010, 20, 983–991 CrossRef CAS.
- Analysis for C8H8ClCu2N4O3 calcd/found (%): C, 25.92/25.72; H, 2.17/2.13, N, 15.11/15.04, Cu, 34.28/33.96, giving satisfactory C, H, N and Cu elemental analyses. IR (KBr, cm−1): 3190, 3120 ν(C–H)ar; 2940, 2920 ν(C–H)al; 1650 [ν(C
C), ν(C
N)]ar; 1400 [δ(C
C), δ(C
N)]ar and 773 γ(
C–H)oop present shifts (in relation with the free ligand) produced by the coordination with copper(II); moreover, the bands at 472, 428 ν(Cu–N); 550, ν(Cu–O); 600 γas(Cu–O) and 356,
322 ν(Cu–Cl) also corroborate the copper coordination. Conductivity (1.02 × 10−3 M in methanol): 32 Ω−1 cm2 mol−1, in agreement with a non-electrolyte complex; UV-vis (1.1 × 10−3 M in methanol) exhibits a single band at 620 nm. - G. Mezei, M. Rivera-Carrillo and R. G. Raptis, Inorg. Chim. Acta, 2004, 357, 3721–3732 CrossRef CAS.
- G. Mezei, R. G. Raptis and J. Telser, Inorg. Chem., 2006, 45, 8841–8843 CrossRef CAS PubMed.
- M. Rivera-Carrillo, I. Chakraborty, G. Mezei, R. D. Webster and R. G. Raptis, Inorg. Chem., 2008, 47, 7644–7650 CrossRef CAS PubMed.
- P. A. Angaridis, P. Baran, R. Boca, F. Cervantes-Lee, W. Haase, G. Mezei, R. G. Raptis and R. Werner, Inorg. Chem., 2002, 41, 2219–2228 CrossRef CAS PubMed.
- M. F. Castello, C. V. Grupioni, R. S. Nunes and J. M. Luiz, J. Therm. Anal. Calorim., 2014, 117, 1145–1150 CrossRef CAS.
- X.-D. Zhang, Z. Zhao, J.-Y. Sun, Y.-Ch. Ma and M.-L. Zhu, Acta Crystallogr., Sect. E: Struct. Rep. Online, 2005, 61, m2643–m2645 CAS.
- H.-D. Wang, Y.-L. Zhou, H.-Y. He, X.-H. Tu and L.-G. Zhu, Acta Crystallogr., Sect. E: Struct. Rep. Online, 2006, 62, m1081–m1082 CAS.
- Traces of oxalic acid were determined by a spectrophotometric method under aerobic conditions after stirring a basic solution of CuCl2 in ethanol. The oxalic acid concentration was determined from the decrease in absorbance of the Cu2+ band and the increase of the Cu–Ox one, changing the colour from blue to yellow.
- J. Su, Y.-Q. Sun, F.-J. Huo, Y.-T. Yang and C.-X. Yin, Analyst, 2010, 135, 2918–2923 RSC.
- M. Arab Chamjangali, L. Sharif-Razavian, M. Yousefi and A. Hossein Amin, Spectrochim. Acta, Part A, 2009, 73, 112–116 CrossRef PubMed.
- O. R. Evans and W. Lin, Cryst. Growth Des., 2001, 1, 9–11 CAS.
- C. S. Hawes and P. E. Kruger, Aust. J. Chem., 2013, 66, 401–408 CrossRef CAS.
- I. Gamba, I. Mutikainen, E. Bouwman, J. Reedijk and S. Bonnet, Eur. J. Inorg. Chem., 2013, 115–123 CrossRef CAS.
- N. Mase, T. Mizumori and Y. Tatemoto, Chem. Commun., 2011, 47, 2086–2088 RSC.
- R. A. Sheldon, Catal. Today, 2015, 247, 4–13 CrossRef CAS.
- M. A. Halcrow, Chem. Soc. Rev., 2013, 42, 1784–1795 RSC.
- J. Park, J.-R. Li, Y.-P. Chen, J. Yu, A. A. Yakovenko, Z. U. Wang, L.-B. Sun, P. B. Balbuena and H.-C. Zhou, Chem. Commun., 2012, 48, 9995–9997 RSC.
- I. H. Hwang, J. M. Bae, W.-S. Kim, Y. D. Jo, C. Kim, Y. Kim, S.-J. Kim and S. Huh, Dalton Trans., 2012, 41, 12759–12765 RSC.
- Y. Zhao, D.-S. Deng, L.-F. Ma, B.-M. Ji and L.-Y. Wang, Chem. Commun., 2013, 49, 10299–10301 RSC.
- T. Zhang, C. Wang, S. Liu, J.-L. Wang and W. Lin, J. Am. Chem. Soc., 2014, 136, 273–281 CrossRef CAS PubMed.
- ESI.†.
- C. M. Reddy, R. C. Gundakaram, S. Basavoju, M. T. Kirchner, K. A. Padmanabhan and G. R. Desiraju, Chem. Commun., 2005, 3945–3947 RSC.
- J. C. Tan, C. A. Merrill, J. B. Orton and A. K. Cheetham, Acta Mater., 2009, 57, 3481–3496 CrossRef CAS.
- C. Malla, G. Reddy, R. Krishnaa and S. Ghosha, CrystEngComm, 2010, 12, 2296–2314 RSC.
- A. Samanta, T. Furuta and J. Li, J. Chem. Phys., 2006, 125, 084714 CrossRef PubMed.
- C. Schütz, J. Sort, Z. Bacsik, V. Oliynyk, E. Pellicer, A. Fall, L. Wågberg, L. Berglund, L. Bergström and G. Salazar-Álvarez, PLoS One, 2012, 7, e45828 Search PubMed.
- M. Guerrero, S. Pané, B. J. Nelson, M. D. Baró, M. Roldán, J. Sort and E. Pellicer, Nanoscale, 2013, 5, 12542–12550 RSC.
Footnotes |
† Electronic supplementary information (ESI) available: X-ray crystallographic file in CIF format for the structure determination of the polymeric Cu(II) complex is enclosed. CCDC 1036962. For ESI and crystallographic data in CIF or other electronic format see DOI: 10.1039/c5ra04028k |
‡ These authors have contributed equally to this work. |
|
This journal is © The Royal Society of Chemistry 2015 |
Click here to see how this site uses Cookies. View our privacy policy here.