DOI:
10.1039/C5RA07647A
(Paper)
RSC Adv., 2015,
5, 57389-57394
Layer-by-layer reduced graphene oxide (rGO)/gold nanosheets (AuNSs) hybrid films: significantly enhanced photothermal transition effect compared with rGO or AuNSs films†
Received
27th April 2015
, Accepted 16th June 2015
First published on 17th June 2015
Abstract
Photothermal materials prepared from graphene and Au nanoparticles have received increasing attentions in various fields ranging from smart devices to advanced medical therapies. In this report, we studied the photothermal transition effects of LbL AuNS/rGO (AuNS: Au nanosheets) films under laser irradiation at 940 nm and comparisons were made with the film of rGO or AuNSs, wherein a single type of photothermal effective species exists. The results indicate that the hybrid LbL AuNS/rGO films displayed enhanced photothermal effects compared with the rGO or AuNSs films. The photothermal performance of the hybrid AuNS/rGO films deteriorated over repeated use or long-term laser irradiation, but the ultimate performance after irradiation was still better than that of the rGO or AuNS films. The hybrid film was able to load the model drug methylene blue (MB), and release MB 4 times faster with NIR irradiation than without, suggesting potential application in the combined chemical and thermal therapies. Thus, the hybrid film of AuNS/rGO is recommended when short-term or few-cycle photothermal applications are required. In comparison, rGO multilayered film better suits long-term or repeated photothermal applications, wherein stable photothermal performance is required.
Introduction
Photothermal transition materials have received remarkably increasing research attentions in cancer therapies, drug delivery, smart devices, and functional films.1–5 Gold nanoparticles and graphene are being especially intensively studied. Gold nanoparticles (AuNPs) present an adjustable surface plasmon resonance (SPR) band and could be programmed to absorb light through the entire visible range as well as the near infrared (IR) range.6 They also serve as signal-enhancing agents in Raman and fluorescence spectra, and thus are extensively used as a multifunctional (sensors, imaging and therapy agents) platform in biomedical devices.7,8 Reduced graphene oxide (rGO), possessing the abilities of both photothermal transition and drug loading, have been engineered into various therapy agents with the aid of chemical surface modification strategies.9–11 In order to combine the advantages of AuNPs and rGO, hybrid materials prepared from both items have received increasing attentions.12,13 For example, graphene-wrapped AuNPs were developed as multimodel cell imaging agents, as well as combined chemo and thermal therapy agents, with enhanced stabilities.14 GO-wrapped surface-enhanced Raman scattering (SERS) tags (gold nanoclusters) served as advanced labeling, photothermal therapy, and monitoring-assistant platforms.15 More interestingly, when the SPR band of AuNPs were tuned to the wavelength of the laser beam, a coupling between the gold nanoparticles and GO, which lead to enhanced photothermal effect, was observed in the hybrid AuNP–GO nanoparticular composites.16,17
Layered films of AuNP/rGO hybrid material, in addition to the hybrid particular composites, also find wide applications as sensors, sample plate as well as matrix in mass spectrometry, electrocatalytic films, and light heating layers in intelligent devices.18–20 Because of the simplicity of the technique, the versatility in the choices of building blocks and the controllability down to the nanoscopic scale, layer-by-layer technique is among the most widely used strategies to prepare hybrid AuNP/rGO films.18–27 Layer-by-layer (LbL) assembled films of AuNP/rGO find potential applications as the versatile coatings of biomedical apparatus or nanoparticles with the abilities of sustained release of drugs and light-induced heating.28 For these reasons, understanding the pros and cons of the hybrid LbL AuNP/rGO film is of paramount importance in the optimization of device performance. However, the systematic study in terms of the photothermal transition effect of LbL AuNP/rGO film is still lacking.
In this report, we prepared LbL AuNP/rGO films and studied their photothermal transition effects. Considering that the photothermal materials are widely used in vivo, the maximum absorbance of our films was adjusted to around 940 nm, which locates in the transparent window (800–1100 nm) of bio tissues. This special maximum absorbance was enabled by carefully choosing the shape of AuNPs, which were nanosheets in the shapes of trigons or hexagons. The hybrid LbL films of these gold nanosheets (AuNSs) and rGO displayed enhanced photothermal effects compared with the film of rGO or AuNSs, wherein a single type of photothermal effective species exists. However, the performance deteriorated over repeated use or long-term laser irradiation for the hybrid film, caused due to the melting of the AuNSs induced by heating. The hybrid film was able to load and release the model drug methylene blue, suggesting potential application in the combined chemical and thermal therapies. With laser irradiation, the amount of the released drug significantly increased. Thus, the hybrid film of AuNS/rGO is recommended when short-term or few-cycle photothermal applications are required. In comparison, rGO multilayered film better suits long-term or repeated photothermal applications, wherein stable photothermal performances are required.
Materials and characterizations
Materials
Graphene oxide (GO) was purchased from Nanjing XFNANO Materials Tech Co., Ltd (XFNANO). Poly(allylamine hydrochloride) (PAH, Mw = 15
000 g mol−1), poly(acrylic acid) (PAA) (Mw = 240
000 g mol−1), and poly(vinyl pyrrolidone) (PVP) (K30, Mw = 40
000 g mol−1) were obtained from Sigma-Aldrich. Hydrogen peroxide (30 wt%) was purchased from Sinopharm Chemical Reagent Co. Ltd. (Beijing, China). Gold chloride (HAuCl3·HCl·4H2O, A.R., Mw = 411.85 g mol−1), sodium borohydride (NaBH4, Mw = 37.95 g mol−1), sodium citrate (Na3C6H5O7·2H2O, Mw = 294.10 g mol−1), and sodium hydroxide (NaOH, Mw = 40.00 g mol−1) were purchased from Beijing Chemical Works (Beijing, China). Gold nanosheets were synthesized as reported and presented absorbance at around 520 nm and 940 nm (Fig. S3†).29–31 Only ultra-pure water and deionized water were used in this study.
Characterizations
UV-visible spectra were obtained using a Hitachi U-3900H spectrophotometer. Zeta-potential values were measured using Zetasizer NanoZS90. Attenuated total reflection Fourier transform infrared (ATR-FTIR) spectra were acquired on an Excalibur 3100 in the range of 4000–600 cm−1 with 4 cm−1 resolution by measuring the absorbance of the films with silicon as the substrate. The surface morphology of the polyelectrolyte multilayers was characterized using atomic force microscopy (AFM; Dimension 3100, Veeco, USA), tapping mode, wherein the scan rate is 1 Hz, 512 lines. The force was optimized for each sample to obtain the minimum noise, and the scan size is indicated by the scale in the images. Scanning electron microscopy (SEM) was measured with a JSM-7600F (Japan). Infrared laser of 940 nm was generated from a laser produced by Beijing Laserwave Optoelectronics Tech. Co., Ltd. The type of the laser source is diode-pumped semiconductor laser. The non-contact temperature meter was purchased from Fluke Corporation, and the instrumental error for temperature measurement is 0.2 °C.
Preparation of PAH-modified rGO
The reduced graphene oxide (rGO) was synthesized by reducing graphene oxide (GO) in the presence of PAH. In detail, GO (0.005 g) was dissolved by an Ultrasonic Cleaner in 20 mL deionized water as the starting material and was placed into 100 mL Erlenmeyer flasks and PAH (pH = 9, 4 mg mL−1, 30 mL) as well as 20 μL hydrazine hydrate was added. The reduction reaction was conducted at 80 degrees for 2 h. After reduction, the synthesized reduced graphene oxide was positively charged.
Fabrication of layer-by-layer self-assembled (LbL) films
Quartz substrates were first cleaned by immersing in piranha solution (vH2SO4/vH2O2 = 3
:
1, caution, corrosive!) for one hour followed by thorough washing with deionized water. Then, the (rGO/AuNS)n films were prepared by dipping the substrates into the rGO/PAH dispersion (0.25 mg mL−1) for 20 min and AuNS dispersion for 20 min alternatively with washing using deionized water for 10 min and drying with N2 in between, until the intended number of layers was obtained. (rGO/PAH)n and (PAH/AuNS)n films were obtained in similar manners with rGO/PAH dispersion or AuNS dispersion being replaced by PAH solution (pH = 9) or PAA solution (pH = 6), respectively.
Photothermal conversion under near-infrared (NIR) light laser irradiation
The photothermal effects of the rGO-modified substrates were studied by measuring the surface temperature of 100 μL water heated by the substrate induced by a NIR laser of 940 nm. The water was carefully added to a container whose bottom was the modified substrate and walls were made of PDMS. A square hole of 7 × 7 mm was opened in a PDMS sheet using a cutter knife. Then, the sheet was pressed against the modified substrate to create the container. Thus, the surface area of the drop of water was fixed by the shape of the container. The NIR laser irradiated from above the water surface from a fixed distance of 5 cm. The power of the laser was set to 0.7 W. The temperature of the water surface was probed by a non-contact infrared temperature measuring instrument. The averages of three data points were used in plotting the heating effect of the multilayers versus irradiation time.
Adsorption and sustained release of methylene blue (MB)
For the sustained-release experiment, substrates covered with (rGO/AuNS)5 films were immersed in a methylene blue solution (0.01 mg mL−1) for 24 hours. Afterwards, these quartz plates were dried with nitrogen. Subsequently, these quartz plates were immersed into phosphate buffered solution (PBS, pH = 7.4) for sustained release. NIR irradiation was applied from a distance of 2.0 cm at a power of 0.7 W if required. The amount of released MB was monitored by UV-VIS spectroscopy every five minutes.
Results and discussion
The synthesized AuNS were a mixture of polygons and spherical particles, as displayed in Fig. S1.† Triangular AuNS were dominant in the mixture, and the edge lengths of the triangles were roughly 100 nm. The thicknesses of the nanosheets were within 10–30 nm range, and a typical height profile of the nanosheet is displayed in Fig. S2.† The Au nanosheets were wrapped by PVP polymers, as indicated by the characteristic FTIR peak of PVP at 1680 cm−1 in Fig. S3.†32 The surface potential of Au nanosheets was −0.5 mV. The prepared AuNS presented surface plasmon resonance at around 520 nm and 940 nm (Fig. S4†). NIR light at around 940 nm was effectively absorbed by the Au plate and this light-absorbance capability can then be used in photothermal transitions.33,34 rGO prepared in our method presented the surface modifications of PAH as indicated by ATR-IR spectra (Fig. S5†)35,36 and a positive surface charge of +45 mV. The AFM image indicated that the thickness of rGO was 4.2 nm (Fig. S6†). Multilayered hybrid films of rGO and AuNSs were prepared using LbL technique. The driving forces for the assembly of the multilayers should be mainly hydrogen bonding, since PVP presents strong capability to form hydrogen bonds because of the strong proton-accepting properties of the carbonyl group in the molecule.37 Electrostatic attraction should also assist the assembly of the multilayers. The sequential deposition of the LbL multilayers was followed by UV-vis spectra (Fig. 1a). The characteristic absorbance of both rGO and AuNSs were clearly observed in the UV-vis spectra. The intensities of the absorbance bands at around 270 nm (corresponding to rGO, Fig. S7†) and at around 520 and 940 nm (corresponding to AuNSs) increased almost linearly with the growth of the number of bilayers. The absorbance band at around 900 nm red-shifted with the increase of the number of bilayers, and the maximum absorbance shifted to approximately 950 nm for the (rGO/AuNS)10 film. The hybrid multilayers absorbed light strongly in the region of near infrared between 850 and 1100 nm. This region is also the transparent window of bio tissues. Thus, the strong absorbance of the film in this region makes it potentially useful in in vivo photothermal transition devices. The multilayers of (PAH/AuNS)n and (PAA/PAH/rGO)n were also constructed in similar manners for comparison. The assembly processes were monitored via UV-vis spectra, and the linear relationship between the absorbance and the number of bilayers was obtained in both cases, indicating the even growth of each bilayer in both cases (Fig. 1b–c). The cross-section of the (rGO/AuNS)5 film was observed using SEM (Fig. 1d), and the image demonstrated that the film surface was scattered by nanoparticles, with dimensions around tens of nanometers. The thickness of (rGO/AuNS)5, (rGO/PAH/PAA)5, and (Au/PAH)5 multilayers are 35.4 nm, 17.9 nm, and 26.4 nm, respectively, measured by AFM (Fig. S8†).
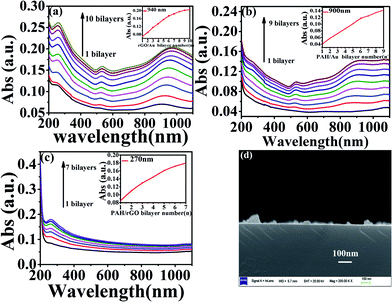 |
| Fig. 1 UV-vis spectra monitoring the layer-by-layer process of (a) (rGO/AuNS)10 (b) (PAH/AuNS)9, and (c) (PAA/PAH/rGO)7 films, showing successful LbL assembly of each type of multilayer. (d) SEM image of cross-section of the (rGO/AuNS)10 film. | |
Since the hybrid multilayered film strongly absorbed near infrared light, we then wondered whether the film could be used as photothermal transition materials. To this end, we fabricated films of (rGO/AuNS)n, (PAA/PAH/rGO)n and (PAH/AuNS)n. For each type of multilayered film, the photothermal transition effect increased with growing number of bilayers (Fig. 2a–c).
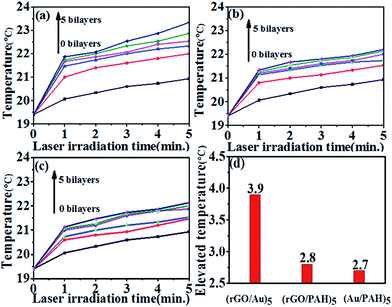 |
| Fig. 2 Photothermal effect indicated by elevated temperature of (a) (rGO/AuNS)5 (b) (PAA/PAH/rGO)5, and (c) (PAH/AuNS)5 films as a function of irradiation time. (d) Substrate temperature of the abovementioned film after an irradiation period of 5 min. | |
After 5 min irradiation, the temperature of the sample increased by 2.6, 2.9, 3.1, 3.5, and 3.9 degrees for the sample on the (rGO/AuNS)n film with n varied from 1 to 5. The temperature increased by 2.1, 2.3, 2.6, 2.7, and 2.8 degrees for the (PAA/PAH/rGO)n film, and by 2, 2.1, 2.5, 2.6, and 2.7 degrees for the (PAH/AuNS)n film, as n varied from 1 to 5. In comparison, the temperature of the sample on the blank quartz substrate increased by only 1.5 degrees. These results demonstrated that all the three types of multilayers presented considerable photothermal effect. These results also demonstrated that given the total cycle of identical assembling, the hybrid (rGO/AuNS)n film significantly outperformed the (PAA/PAH/rGO)n or (PAH/AuNS)n films. For example, the temperature increase was 3.9 degrees for the (rGO/AuNS)5 film, but only 2.8 degrees and 2.7 degrees for the (PAA/PAH/rGO)5 or (PAH/AuNS)5 films (Fig. 2d).
Subsequently, we wondered whether the hybrid film presents a higher transition effect compared with the (PAA/PAH/rGO)n or (PAH/AuNS)n films, wherein only one type of photothermal effective component exists. To this end, we fabricated films of (rGO/AuNS)1, (PAH/AuNS)2 and (PAA/PAH/rGO)2. In these films, two layers (referred as two cycles of deposition in LbL assembly, although the accurate weight in each cycle for different species may differ) of photothermal effective species (rGO or AuNS) were assembled. The hybrid film induced the largest temperature increase when the IR irradiation duration was longer than 2 min. After 5 min irradiation, the temperature of the sample rose by 2.6 degrees for the hybrid film. (PAA/PAH/rGO)2 film induced a medium temperature increase of 2.3 degrees, and (PAH/AuNS)2 film induced the minimum temperature increase of 2.1 degrees (Fig. 3a). Further increase of the number of bilayers presented a similar trend, verified by comparison of the photothermal effects of (rGO/AuNS)2, (PAA/PAH/rGO)4 and (PAH/AuNS)4 films (Fig. 3b). The (rGO/AuNS)2 film induced the largest temperature increase of 2.9 degrees, the (PAA/PAH/rGO)4 film, medium of 2.7 degrees, and the (PAH/AuNS)4 film, the least of 2.6 degrees. These results indicated that in the assembly process of LbL, the combination of AuNS and rGO gave a synergistic effect. The hybrid film outperformed the films constructed using a single effective component, be it rGO or AuNS, provided that the number of layers of the effective components were identical.
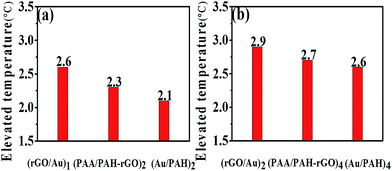 |
| Fig. 3 Substrate temperature after an irradiation period of 5 min for the (a) (rGO/AuNS)1, (PAH/AuNS)2 and (PAA/PAH/rGO)2 and (b) (rGO/AuNS)2, (PAA/PAH/rGO)4 and (PAH/AuNS)4 films. | |
However, further study revealed that the hybrid (rGO/AuNS)5 film, effective in short-term photothermal transition experiments, suffered inconveniences in long-term or repeated applications. The absorbance of AuNS gradually diminished with the elongation of the irradiation period, and after 60 min irradiation, the absorbance at around 520 nm and 940 nm, which were characteristic of the AuNSs disappeared completely (Fig. 4a).
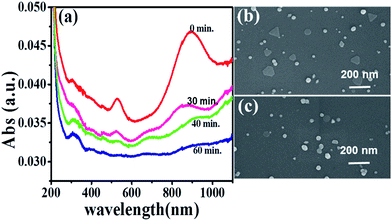 |
| Fig. 4 UV-vis spectra of (rGO/AuNS)5 film after infrared irradiation periods of 0, 30, 40, and 60 min. SEM images of the substrate surfaces (b) before and (c) after infrared irradiation of 60 min. | |
While the absorbance characteristic of rGO still existed, SEM images supported the deterioration of AuNS with irradiation. A typical SEM image of the multilayer surface is displayed in Fig. 4b. AuNS and Au nanoparticles co-existed on the substrate surface before irradiation. In contrast, after cumulative irradiation for 50 min, AuNS largely disappeared on the surface and only quasi-spherical particles were observed.
Because of the deterioration of AuNS with irradiation, it is thus important to study the performance stability of the multilayers with repeated irradiation. The substrates of (rGO/AuNS)5, (PAA/PAH/rGO)5 and (PAH/AuNS)5 films were then repeatedly irradiated and cooled down for over 10 cycles, wherein the total irradiation time lasted over 50 min (5 min irradiation for each cycle, Fig. 5). The hybrid film presented the highest temperature increase during each cycle and the temperature increase shrank with the repetition of cycles. About 15% of deterioration was observed after 10 cycles, but the temperature increase was still larger than that of the (PAA/PAH/rGO)5 and (PAH/AuNS)5 films. (PAA/PAH/rGO)5 films presented stable heating performance of an increase of 2.7 degrees for each cycle.
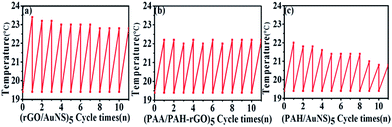 |
| Fig. 5 Substrate temperatures for cycles of 5 min heatings with coolings in between for the (a) (rGO/AuNS)5 (b) (PAA/PAH/rGO)5, and (c) (PAH/AuNS)5 films. | |
The heating performance of (PAH/AuNS)5 films was the poorest and deteriorated most rapidly over repeated heating. About 54% decrease of temperature rise was observed after 10 cycles of heating. These results demonstrated that (rGO/AuNS)5 films presented the most effective photothermal transition in relatively few repetition cycles, e.g., 10 cycles. However, the deterioration of the photothermal effect should be considered, especially in long-term and repetitive uses.
In order to explore whether the hybrid (rGO/AuNS)5 film (1.3 × 2.5 cm2, both sides of the quartz substrate were covered by the film) effectively heated up a relatively large amount of water, we immersed the substrate into a vial that contained 10 mL water. After irradiation for 10 min, the temperature of the water increased from 19.4 degrees to 22.2 degrees. This result demonstrated that the film, although as thin as less than 100 nm, effectively warmed up the surrounding environment.
In the last step, we explored the loading and release capacities of the hybrid film (rGO/AuNS)5 (Fig. 6 & S9†). The film was able to load MB by passive infiltration. Immersed in PBS, the loaded MB gradually diffused out into the solution. It can be noted that irradiation remarkably increased the released amount of MB. With irradiation, a large proportion of MB (78% of the maximum released amount) was released quickly in the first 20 min. Then, the release continued in a slower manner. Around 70 min, the released amount of MB reached a plateau and no longer increased. In the meantime, the temperature of the solutions (3 mL) increased by only approximately 2 degrees with irradiation. In clear comparison, the substrate without irradiation released much less MB, whose amount was only 22% the amount released under irradiation. This clear comparison between the release capability with and without irradiation demonstrated that the hybrid multilayer only released small molecules in effective manners under irradiation. Thus, the film would have potential applications as molecule reservoirs. The small molecules were kept largely in the film and were only able to be effectively released under IR trigger at intended occasions. The release profile of the hybrid film demonstrated that controlled release could be obtained with the hybrid (rGO/AuNS)5 film. The high controllability suggests that the films could be used as drug reservoirs and function in an induced-release manner, and thus present low toxicity to the unintended area of the body if used in vivo.
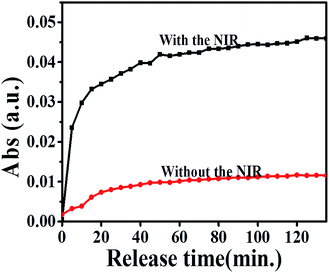 |
| Fig. 6 Release profile of MB from the (rGO/AuNS)5 film with and without NIR irradiation, showing that only with irradiation was the MB effectively released from the multilayered films. The absorbance intensity at 664 nm was used in the plotting. | |
Conclusion
In this report we studied the photothermal effect of the hybrid (rGO/AuNS)n film and discussed the pros and cons of the hybrid films in comparison with the single- (photothermal effective) component film of (PAA/PAH/rGO)n and (PAH/AuNS)n. For all three types of films, the photothermal effect increased with growing number of assembly. The hybrid films presented the highest photothermal effect compared with (PAA/PAH/rGO)n and (PAH/AuNS)n films with identical cycles of assembly. However, because of the instability of AuNS with irradiation, the photothermal effect of hybrid (rGO/AuNS)n films deteriorated over time, and about 15% deterioration was observed over 10 cycles of repeated heating and cooling with the heating duration being 5 min for each cycle, but the ultimate heating effect was still higher than the (PAA/PAH/rGO)n and (PAH/AuNS)n films. The hybrid (rGO/AuNS)5 films released model drug MB in a controlled manner adjusted by IR irradiation. With IR irradiation, 78% of the maximum released amount of MB was effectively released within 20 min. However, without irradiation, only 22% of MB was released compared with irradiation. The report demonstrated that the hybrid (rGO/AuNS)n film serves as an effective heating film with drug loading and controlled release properties. We expect this report to promote the rational design and selection of graphene- or AuNS-based photothermal materials in smart devices or medical therapy materials.
Acknowledgements
This study was supported by the NSFC (21303169, 51273030), the Fundamental Research Funds for the Central Universities (2652013115, DUT14ZD217), the Beijing Nova Program (Z141103001814064), the Beijing Specific Project to Foster Elitist (2013D009015000001), the National High Technology Research and Development Program of China (863 Program 2012AA06A109) and the special co-construction project of Beijing city education committee.
Notes and references
- V. Shanmugam, S. Selvakumar and C. S. Yeh, Chem. Soc. Rev., 2014, 43, 6254 RSC.
- S. Sortino, J. Mater. Chem., 2012, 22, 301 RSC.
- C. A. Tao, X. Zou, Z. Hu, H. Liu and J. Wang, Polym. Compos., 2014, 19 DOI:10.1002/pc.23303.
- Y. W. Chen, P. J. Chen, S. H. Hu, I. W. Chen and S. Y. Chen, Adv. Funct. Mater., 2014, 24, 451 CrossRef CAS PubMed.
- A. Bansal and Y. Zhang, Acc. Chem. Res., 2014, 47, 3052 CrossRef CAS PubMed.
- J. J. Qiu and W. D. Wei, J. Phys. Chem. C, 2014, 118, 20735–20749 CAS.
- X. F. Li, C. Y. Chen, Y. H. Zhao and X. Y. Yuan, Prog. Biochem. Biophys., 2014, 41, 739 CAS.
- W. Xie and S. Schlucker, Rep. Prog. Phys., 2014, 77, 116502 CrossRef PubMed.
- H. Kim, D. Lee, J. Kim, T. I. Kim and W. J. Kim, ACS Nano, 2013, 7, 6735 CrossRef CAS PubMed.
- C. A. Tao, J. F. Wang, S. Q. Qin, Y. A. Lv, Y. Long, H. Zhu and Z. H. Jiang, J. Mater. Chem., 2012, 22, 24856 RSC.
- S. X. Shi, F. Chen, E. B. Ehlerding and W. B. Cai, Bioconjugate Chem., 2014, 25, 1609 CrossRef CAS PubMed.
- R. K. Shervedani and A. Amini, Electrochim. Acta, 2014, 121, 376 CrossRef CAS PubMed.
- S. Z. Nergiz, N. Gandra, S. Tadepalli and S. Singamaneni, ACS Appl. Mater. Interfaces, 2014, 6, 16395 CAS.
- X. Bian, Z. L. Song, Y. Qian, W. Gao, Z. Q. Cheng, L. Chen, H. Liang, D. Ding, X. K. Nie, Z. Chen and W. H. Tan, Sci. Rep., 2014, 4, 6093 CrossRef CAS PubMed.
- D. H. Lin, T. Q. Qin, Y. Q. Wang, X. Y. Sun and L. X. Chen, ACS Appl. Mater. Interfaces, 2014, 6, 1320 CAS.
- A. F. Zedan, S. Moussa, J. Terner and G. Atkinson, ACS Nano, 2013, 7, 627 CrossRef CAS PubMed.
- D. K. Lim, A. Barhoumi, R. G. Wylie, G. Reznor, R. S. Langer and D. S. Kohane, Nano Lett., 2013, 13, 4075 CrossRef CAS PubMed.
- Y. Wang, R. Yuan, Y. Q. Chai, Y. L. Yuan and L. J. Bai, Biosens. Bioelectron., 2012, 38, 50 CrossRef CAS PubMed.
- Y. Choi, M. Gu, J. Park, H. K. Song and B. S. Kim, Adv. Energy Mater., 2012, 2, 1510 CrossRef CAS PubMed.
- T. R. Kuo, D. Y. Wang, Y. C. Chiu, Y. C. Yeh, W. T. Chen, C. H. Chen, C. W. Chen, H. C. Chang, C. C. Hu and C. C. Chen, Anal. Chim. Acta, 2014, 809, 97 CrossRef CAS PubMed.
- Y. Li, X. Wang and J. Q. Sun, Chem. Soc. Rev., 2012, 41, 5998 RSC.
- M. J. Cheng, F. Shi, J. S. Li, Z. F. Lin, C. Jiang, M. Xiao, L. Q. Zhang, W. T. Yang and T. S. Nishi, Adv. Mater., 2014, 26, 3009 CrossRef CAS PubMed.
- J. S. Park, S. M. Cho, W. J. Kim, J. Park and P. J. Yoo, ACS Appl. Mater. Interfaces, 2011, 3, 360 CAS.
- Y. Zhou, M. J. Cheng, X. Q. Zhu, Y. J. Zhang, Q. An and F. Shi, ACS Appl. Mater. Interfaces, 2013, 5, 8308 CAS.
- K. Ariga, J. P. Hill and Q. M. Ji, Phys. Chem. Chem. Phys., 2007, 9, 2319 RSC.
- Q. An, Y. Zhou, Y. J. Zhang, Y. H. Zhang and F. Shi, RSC Adv., 2014, 4, 5683 RSC.
- M. J. Cheng, Q. Liu, Y. M. Xian and F. Shi, ACS Appl. Mater. Interfaces, 2014, 6, 7572 CAS.
- K. Ariga, M. McShane, Y. M. Lvov, Q. M. Ji and J. P. Hill, Drug Delivery, 2011, 8, 633 CAS.
- X. K. Liu, H. L. Xu, H. B. Xia and D. Y. Wang, Langmuir, 2012, 28, 13720 CrossRef CAS PubMed.
- P. Jiang, J. J. Zhou, R. Li, Y. Gao, T. L. Sun, X. W. Zhao, Y. J. Xiang and S. S. Xie, J. Nanopart. Res., 2006, 8, 927 CrossRef CAS.
- T. Tang and I. W. Hamley, Colloids Surf., A, 2009, 336, 1 CrossRef CAS PubMed.
- K. Chan, L. E. Kostun, W. E. Tenhaeff and K. K. Gleason, Polymer, 2006, 47, 6941 CrossRef CAS PubMed.
- A. J. Mieszawska, W. J. M. Mulder, Z. A. Fayad and D. P. Cormode, Mol. Pharmaceutics, 2013, 10, 831 CrossRef CAS PubMed.
- K. Park, H. Koerner and R. A. Vaia, Nano Lett., 2010, 10, 1433 CrossRef CAS PubMed.
- V. Zucolotto, M. Ferreira, M. R. Cordeiro, C. J. L. Constantino, D. T. Balogh, A. R. Zanatta, W. C. Moreira and O. N. Oliveira, J. Phys. Chem. B, 2003, 107, 3733 CrossRef CAS.
- C. Bonazzola and E. J. Calvo, Langmuir, 2003, 19, 5279 CrossRef CAS.
- S. A. Sukhishvili and S. Granick, Macromolecules, 2002, 35, 301 CrossRef CAS.
Footnote |
† Electronic supplementary information (ESI) available: SEM, AFM images and UV-vis spectra of AuNS, and UV-vis spectra of rGO. See DOI: 10.1039/c5ra07647a |
|
This journal is © The Royal Society of Chemistry 2015 |
Click here to see how this site uses Cookies. View our privacy policy here.