Hybrid material based on a coordination-complex-modified polyoxometalate nanorod (CC/POMNR) and PPy: a new visible light activated and highly efficient photocatalyst†
Received
25th September 2014
, Accepted 28th October 2014
First published on 12th November 2014
Abstract
To improve the photocatalytic activity of a coordination-complex-modified polyoxometalate (CC/POM), a new type of hybrid material (abbreviated as PPy/CC/POMNR) was fabricated by the combination of its nanorod (CC/POMNR) and polypyrrole (PPy) via a facile in situ chemical oxidation polymerization process under the initiation of ammonium persulfate (APS). Under the irradiation of visible light, PPy/CC/POMNR exhibited higher photocatalytic activity compared to CC/POMNR, PPy, and their mechanically blended products formed on the degradation of Rhodamine B (RhB). Optical and electrochemical tests showed that the enhancement of photocatalytic performance can be attributed to the high separation efficiency of the photogenerated electrons and holes on the interface of CC/POMNBs and PPy, which results from the synergistic effect between them. Furthermore, the influence of the concentration ratio between pyrrole (Py) and APS on the morphology, conductivity, and photocatalytic properties of the PPy/CC/POMNR is discussed and the optimal condition to fabricate a hybrid material with high efficiency was determined. These results suggest that the hybrid of CC/POMNR and PPy would be a feasible strategy to enhance the photocatalytic activity of CC/POMNR.
Introduction
Recently, serious environmental problems caused by organic dyes have accelerated the development of efficient methods for their treatment.1 In this aspect, photocatalytic degradation has been proven to be a feasible method to decompose these pollutants into less dangerous matter.2 Because of its cleanness and low cost in the organic dyes treatment process, CC/POM, especially nanoscale coordination-complex-modified polyoxometalate (NCC/POM), has attracted significant interest from researchers.3 Compared with other photocatalysts, the stability of NCC/POM is considerably higher, which makes it more convenient for recovery and recycling.4 Furthermore, because of its large surface area, NCC/POM can contact with organic dyes and promote a more complete decomposition.5 However, several drawbacks, such as being inactive in the visible light region (due to the wide band gap of NCC/POM), as well as low photocatalytic efficiency (due to the rapid recombination of photogenerated electrons and holes during the photocatalysis process), still impede the further application of NCC/POM in wastewater treatment.6,7 Now, the extension of the photoresponse region from ultraviolet to the visible light region and the enhancement of photocatalytic efficiency have become significant problems in the application of NCC/POM photocatalysts.
To resolve these problems, fabrication of a hybrid of NCC/POM with a visible light active material, which possesses excellent photogenerated electron–hole pair separation properties, is considered as a feasible strategy. In this context, PPy is an ideal choice. First, its photoresponse region is very broad, ranging from ultraviolet to the visible light region.8 Second, as an excellent photogenerated hole transporting material, PPy can effectively separate electron–hole pairs and impede a recombination of the electron and hole again, which can enhance the photocatalytic efficiency.9 More importantly, the polymerization condition for PPy is mild, which prevents the decomposition of NCC/POM during fabrication of the hybrid material.10 To date, PPy has been applied to enhance the photocatalytic property of some typical photocatalysts, such as TiO2 and ZnO, and has received very favorable results.11–15 Encouraged by these, we want to improve the photocatalytic property of NCC/POM through its hybridization with PPy.
Our ideas were confirmed as reasonable by studies into the visible light active photocatalyst PPy/ZnP2Mo5NR, which was synthesized by the hybridization of PPy and nanorods in a new CC/POM, {[Zn(PyBim)2(H2O)(P2Mo5O23)]·(H2PyBim)2·(H2O)5}n (ZnP2Mo5, PyBim = 2-(3-pyridyl)benzimidazole). The photocatalytic degradation of RhB was investigated and the results indicated that the hybrid of ZnP2Mo5NR and PPy effectively enhanced its photocatalytic property. During the polymerization of PPy, the concentration ratio between Py and APS (abbreviated as [Py]/[APS]) had a great effect on its chemical and physical properties, which may further influence the photocatalytic properties of the resulting PPy/ZnP2Mo5NR hybrid material.16 To test this, an in situ chemical oxidative polymerization of Py was conducted under different conditions and an optimal[Py]/[APS] value to obtain PPy/ZnP2Mo5NR with excellent photocatalytic activity was obtained Table 1.
Table 1 Degradation efficiency of RhB with different photocatalysts
Photocatalyst |
Condition |
Time (hours) |
η (%) |
ZnP2Mo5NR
|
Ultraviolet light irradiation |
8 |
50.07 |
ZnP2Mo5NR
|
Visible light irradiation |
8 |
0.01 |
PPy(A)/ZnP2Mo5NR
|
Visible light irradiation |
2 |
76.12 |
PPy(B)/ZnP2Mo5NR
|
Visible light irradiation |
2 |
73.92 |
PPy(C)/ZnP2Mo5NR
|
Visible light irradiation |
2 |
86.92 |
PPy(D)/ZnP2Mo5NR
|
Visible light irradiation |
2 |
60.86 |
PPy(A)
|
Visible light irradiation |
6 |
59.07 |
PPy(B)
|
Visible light irradiation |
6 |
40.12 |
PPy(C)
|
Visible light irradiation |
6 |
35.82 |
PPy(D)
|
Visible light irradiation |
6 |
30.48 |
PPy(A)/ZnP2Mo5NR(M)
|
Visible light irradiation |
6 |
50.85 |
PPy(B)/ZnP2Mo5NR(M)
|
Visible light irradiation |
6 |
61.99 |
PPy(C)/ZnP2Mo5NR(M)
|
Visible light irradiation |
6 |
64.72 |
PPy(D)/ZnP2Mo5NR(M)
|
Visible light irradiation |
6 |
43.20 |
Experimental section
Materials and synthesis
All the purchased chemicals were of reagent grade and used without further purification. Elemental analyses (C, H, and N) were performed on a Perkin-Elmer 2400 CHN Elemental Analyzer. P, Mo, and Zn were determined with a Leeman inductively coupled plasma (ICP) spectrometer. The morphology was observed on an ultra plus field emission scanning electron microscope (ZEISS, Germany). PXRD patterns were recorded on D8 X-ray diffractometer, employing monochromatized Cu Kα incident radiation. FTIR spectra were recorded in the range of 4000–400 cm−1 on an Alpha Centaur FTIR spectrophotometer using KBr pellets. Diffuse reflectance spectra (DRS) were recorded on a Shimadzu-2501 PC spectrometer using BaSO4 as a standard. Conductivity measurements were performed by the conventional four-probe technique. Electrochemical experiments were conducted on a CHI 660E electrochemical workstation. UV-visible adsorption spectra were recorded using a Hitachi U-3010 UV-visible spectrometer.
Synthesis of {[Zn(PyBim)2(H2O)(P2Mo5O23)]·(H2PyBim)2·(H2O)5}n(Z nP2Mo5)
ZnP2Mo5 was prepared from a mixture of Zn(OAc)2·2H2O (0.022 g, 0.1 mmol), PyBim (0.019 g, 0.1 mmol), Na2MoO4·2H2O (0.048 g, 0.2 mmol), H3PO4 (0.1 mL), and 5 mL H2O. The mixture was stirred for 10 min, and then transferred to a 15 mL Teflon-lined stainless steel bomb and maintained at 180 °C under autogenous pressure for 4 days. The reaction system was cooled to room temperature over 24 h. A large amount of yellow crystals of ZnP2Mo5 were obtained. Yield: 81% (based on Zn). Anal. calcd for C48H52N12O29ZnP2Mo5: C, 30.86%; H, 2.81%; N, 8.99%; P, 3.32%; Mo, 25.68%; Zn, 3.50%. Found: C, 30.73%; H, 2.92%; N, 9.03%; P, 3.42%; Mo, 25.54%; Zn, 3.38%.
Synthesis of ZnP2Mo5NR
Crystals of ZnP2Mo5 were ground for 4 h with an agate mortar and pestle. The resulting powder was dissolved in methanol and placed in a Teflon autoclave, which was heated in a microwave oven at 400 W for 2 h. The resulting ZnP2Mo5NR was separated by centrifugation, rinsed with water, and then dried in a vacuum at 70 °C for 24 h.
Synthesis of PPy/ZnP2Mo5NR hybrid material
Py (0.034 g, 5 × 10−4 mol) was dissolved in 20 mL H2O. ZnP2Mo5NR (4.671 g, 2.5 × 10−3 mol) was also placed in the above solution and supersonically dispersed for 25 min. 10 mL APS solution was slowly added to the above mixture as oxidant. The product was stirred for 20 min, and then left undisturbed for 12 h. The resulting PPy/ZnP2Mo5NR hybrid material was separated, subsequently rinsed with water, alcohol and finally dried at 60 °C for 24 h in an oven. In the experiment, hybrid material was prepared in the ratio of [Py]
:
[APS] of 4
:
1, 2
:
1, 1
:
1, and 1
:
2. The products were labeled as PPy(A)/ZnP2Mo5NR, PPy(B)/ZnP2Mo5NR, PPy(C)/ZnP2Mo5NR and PPy(D)/ZnP2Mo5NR, respectively.
Synthesis of PPy and PPy/ZnP2Mo5NR(M)
PPy(A) to PPy(D) were obtained using a similar process to PPy/ZnP2Mo5NR hybrid material, except that ZnP2Mo5NR was not added. PPy(A)/ZnP2Mo5NR(M) to PPy(D)/ZnP2Mo5NR(M) were synthesized by the directly mixing of PPy and ZnP2Mo5NR.
Photocatalytic activity study
The photocatalytic activities of the samples were evaluated by the degradation of RhB in the aqueous solution. 80 mL RhB aqueous solution with a concentration of 10−5 M was mixed with 20 mg of catalyst, which was exposed to illumination. Before turning on the lamp, the suspension containing RhB and the photocatalyst was magnetically stirred in the dark for 40 min until an adsorption–desorption equilibrium was established. Then, the samples were regularly taken out from the reactor and immediately centrifuged for the separation of any suspended solid. The transparent solution was analyzed by a UV-vis spectrometer. A 300 W medium pressure mercury lamp served as an ultraviolet light source and a 300 W Xe lamp with a cutoff filter (λ ≥ 420 nm) served as a visible light source.
Electrochemical measurements
Photoelectrochemical tests were carried out with a conventional three-electrode system in a quartz cell filled with 0.1 M Na2SO4 electrolyte (100 mL). The ZnP2Mo5NR/ITO or PPy/ZnP2Mo5NR/ITO electrodes served as the working electrode. The counter and reference electrodes were a Pt plate and a saturated calomel electrode (SCE), respectively. A 300 W Xe lamp with a cutoff filter (λ ≥ 420 nm) were used as the excitation light source for visible light irradiation. Electrochemical impedance spectra (EIS) were recorded in the potentiostatic mode. The amplitude of the sinusoidal wave was 10 mV, and the frequency range of the sinusoidal wave was from 100 kHz to 0.05 Hz.
X-ray crystallography
A suitable single crystal of ZnP2Mo5 was carefully selected under an optical microscope and glued onto glass fiber. Structural measurements were performed on a Bruker AXS SMART APEX II CCD diffractometer at 293 K. The structure was solved by the direct method and refined by the full-matrix least-squares method on F2 using the SHELXTL 97 crystallographic software package.17 Anisotropic thermal parameters were used to refine all the non-hydrogen atoms. Carbon-bound hydrogen atoms were placed in geometrically calculated positions; oxygen-bound hydrogen atoms were located in the difference Fourier maps and then refined with isotropic temperature factors. The X-ray structural analysis results are listed in Table S1.† Selected bond lengths are listed in Table S2.† Selected bond angles are listed in Table S3.†
Structure and morphology study
Single crystal X-ray analysis reveals that the fundamental unit of ZnP2Mo5 is made up of one Strandberg-type polyanion [P2Mo5O23]6– (abbreviated as P2Mo5), one Zn(II) cation, two PyBim ligands, and one coordinated water molecule. The P2Mo5 cluster is similar to the Strandberg-type polyanion in other CC/POMs, which can be described as a ring of five distorted MoO6 octahedrons with two PO4 tetrahedrons capped on each side.18 Each phosphate subunit shares three oxo-groups with the molybdate ring, whereby one of these oxo-groups adopts the μ2-bridging mode, whereas the other two adopt the μ3-bridging mode. In this P2Mo5 cluster, the Mo–Ob bond distances range from 2.210(5) to 2.425(5) Å; the Mo–Oc bond distances are in the range of 1.863(5) to 1.961(5) Å and the Mo–Od bond distances range from 1.698(5) to 1.731(6) Å. The P–O bond distances vary from 1.522(5) to 1.568(5) Å, and the O–P–O bond angles are in the range of 106.4(3)° to 112.6(3)°. The adjacent P2Mo5 clusters are connected by a [Zn(PyBim)2(H2O)] unit with Zn1–O1 = 1.980(5) Å and Zn1–O22 = 1.982(5) Å, which results in the 1D chain-like structure of ZnP2Mo5 (Fig. 1a). The morphology of ZnP2Mo5NR was studied with SEM. The surface of ZnP2Mo5NR is very smooth, its length and width both range from 60 nm to 80 nm, whereas its height varies from 0.8 μm to 1.5 μm (Fig. 1b).
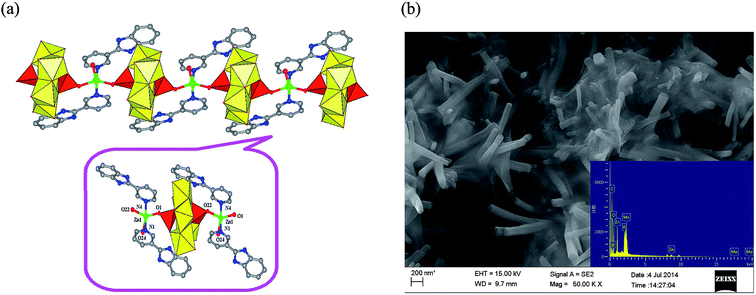 |
| Fig. 1 (a) 1D chain structure of ZnP2Mo5 (inset, the fundamental unit of ZnP2Mo5); (b) SEM picture of ZnP2Mo5NR (inset, EDX of ZnP2Mo5NR). | |
The morphologies of the PPy/ZnP2Mo5NR hybrid materials were also studied with SEM. It can be clearly observed that PPy particles are evenly located around ZnP2Mo5NR. In hybrid materials, the dimensions and elemental contents of ZnP2Mo5NR are similar with those of pure ZnP2Mo5NR, which shows that its morphology and structure are not altered during the polymerization process of Py. As [Py]/[APS] = 4, ZnP2Mo5NR are surrounded by PPy nanoparticles with diameters of about 30 to 40 nm (Fig. 2a). If the [Py]/[APS] value decreases, in addition to these small PPy particles, some large PPy nanoparticles with diameters of 200 to 300 nm appear around ZnP2Mo5NR (Fig. 2b and c). When [Py]/[APS] = 0.5, small PPy particles completely disappear, and then only PPy particles with large sizes are left (Fig. 2d). The alteration of morphology can be attributed to the influence of the [Py]/[APS] value. When [Py]/[APS] = 4, [APS] is insufficient, and this leads to a slow polymerization of Py, which results in PPy with a small size. As [APS] increases, the polymerization process is speeded up, which leads to the secondary polymerization of Py on early formed PPy, which usually results in PPy particles with larger sizes.
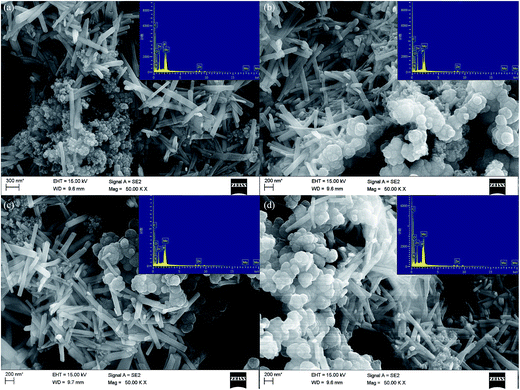 |
| Fig. 2 SEM picture of PPy/ZnP2Mo5NR (inset, EDX of PPy/ZnP2Mo5NR); (a) PPy(A)/ZnP2Mo5NR; (b) PPy(B)/ZnP2Mo5NR; (c) PPy(C)/ZnP2Mo5NR; (d) PPy(D)/ZnP2Mo5NR. | |
PXRD was employed to study the structures of ZnP2Mo5NR and PPy/ZnP2Mo5NR (Fig. 3a and b). ZnP2Mo5NR and PPy/ZnP2Mo5NR adopt similar diffraction patterns with ZnP2Mo5, which shows that in ZnP2Mo5NR and PPy/ZnP2Mo5NR the structures of ZnP2Mo5 are still retained. Furthermore, the peaks belonging to PPy are not observed, which indicates that the amount of PPy may be too small to determine its existence.19 In these hybrid materials, to study the interactions between ZnP2Mo5NR and PPy, FTIR spectra were employed. In ZnP2Mo5NR, the characteristic bands at 818, 921 and 1052 cm−1 can be attributed to the stretching of Mo–Ob–Mo, Mo–Od and P–Oa, respectively.20 As for PPy/ZnP2Mo5NR, the peaks move to a higher wavenumber region (Fig. 3c and d). On the contrary, the N–H stretching of PPy moves to a lower wavenumber region compared with pure PPy. All these movements reveal the effective interactions between ZnP2Mo5NR and PPy, which can be attributed to the existence of hydrogen bonds and π–π interactions.21
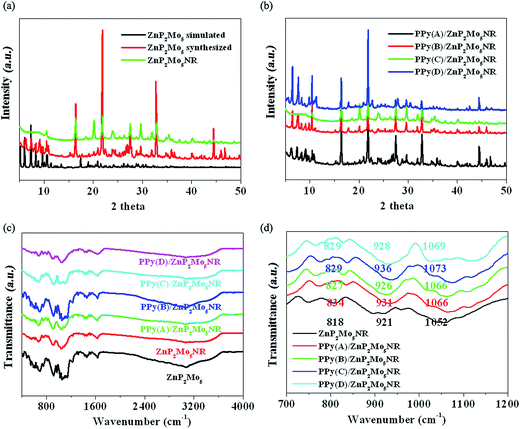 |
| Fig. 3 (a) PXRD of ZnP2Mo5 and ZnP2Mo5NR; (b) PXRD of PPy/ZnP2Mo5NR; (c) FTIR of ZnP2Mo5NR and PPy/ZnP2Mo5NR; (d) Enlarged FTIR of ZnP2Mo5NR and PPy/ZnP2Mo5NR. | |
Optical properties
The UV-vis diffuse reflectance spectra (DRS) of ZnP2Mo5NR and PPy/ZnP2Mo5NR hybrid materials were studied. Compared with visible light inactive ZnP2Mo5NR, PPy/ZnP2Mo5NR hybrid materials show a strong photoresponse in the ultraviolet and visible light region, which suggests PPy is a suitable option to extend the photoresponse region of ZnP2Mo5NR (Fig. 4a). To study the influences of the ratio of [Py]
:
[APS] on the photoresponse region in detail, band gaps (Eg) of ZnP2Mo5NR and PPy/ZnP2Mo5NR hybrid materials were obtained from Tauc's equation (Fig. 4b). We determine that with a decrease in the ratio of [Py]
:
[APS], Eg of the hybrid material becomes narrower. If the ratio of [Py]
:
[APS] continuously decreases, the opposite occurs. In these hybrid materials, PPy(C)/ZnP2Mo5NR possesses the narrowest band gap, which indicates that the ratio of [Py]
:
[APS] = 1 is the optimal condition to fabricate hybrid material with a more extensive photoresponse region.
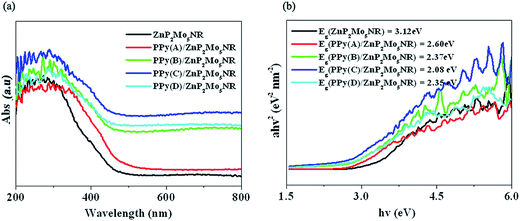 |
| Fig. 4 (a) DRS of ZnP2Mo5NR and PPy/ZnP2Mo5NR; (b) Tauc plots ZnP2Mo5NR and PPy/ZnP2Mo5NR. | |
Electrochemical analysis
The interface charge separation efficiency can be investigated by photocurrent spectra and electrochemical impedance spectroscopy (EIS). Photocurrent responses were conducted under the irradiation of ultraviolet and visible light. We can clearly observe that the ZnP2Mo5NR/ITO electrode only exhibits a photocurrent response under ultraviolet light (Fig. 5a). On the contrary, PPy/ZnP2Mo5NR/ITO electrodes show a strong photocurrent response under both ultraviolet and visible light irradiation. Their photocurrents both rapidly decrease to zero when the light is switched off (Fig. 5b). This indicates that the hybrid of PPy and ZnP2Mo5NR can effectively enhance its photocurrent. Furthermore, with the increasing ratio of [Py]
:
[APS], the photocurrent of the PPy/ZnP2Mo5NR/ITO electrodes increase at first, and then decrease. The PPy(C)/ZnP2Mo5NR/ITO electrode exhibited the largest photocurrent.
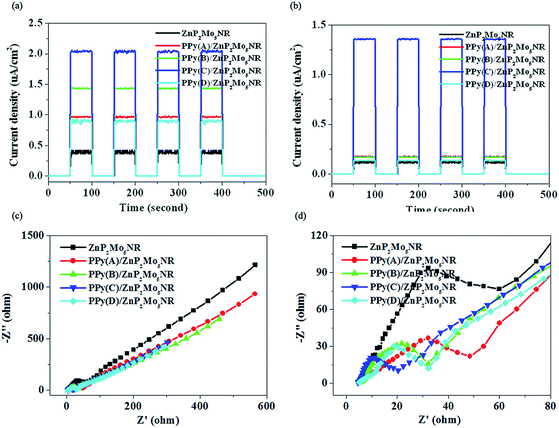 |
| Fig. 5 (a) Photocurrent spectra of ZnP2Mo5NR and PPy/ZnP2Mo5NR under ultraviolet light; (b) photocurrent spectra of ZnP2Mo5NR and PPy/ZnP2Mo5NR under visible light. (c) Nyquist plots of ZnP2Mo5NR and PPy/ZnP2Mo5NR electrodes under visible light. (d) Enlarged Nyquist plots of ZnP2Mo5NR and PPy/ZnP2Mo5NR electrodes. | |
To study the charge separation and transfer process in detail, EIS was employed (Fig. 5c and d). In EIS, the radius of the arc on the Nyquist plot reflects the reaction rate on the surface of the electrode.22 The arc radius of all the PPy/ZnP2Mo5NR/ITO electrodes are smaller than for the ZnP2Mo5NR/ITO electrode, which indicates a more effective separation of the photogenerated electron–hole pair, as well as a faster interfacial charge transfer, after the introduction of PPy. In the hybrid material electrodes, the PPy(C)/ZnP2Mo5NR/ITO electrode exhibits the smallest arc radius, which indicates that it possesses the highest photogenerated charge separation efficiency.
In summary, these electrochemical tests matched well with the studies of optical properties. All these results indicate that the hybrid of PPy and ZnP2Mo5NR decreases the recombination of the photogenerated electron–hole pair. Furthermore, compared with other values, the ratio of [Py]
:
[APS] = 1 is a more appropriate condition to fabricate hybrid materials with excellent electron–hole pair separation efficiency.
Photocatalytic property studies
The photocatalytic activities of ZnP2Mo5NR and PPy/ZnP2Mo5NR hybrid materials were evaluated by the degradation of RhB in aqueous solution. Like other CC/POM photocatalysts, as an ultraviolet light active photocatalyst, ZnP2Mo5NR exhibited no effect on RhB in the visible light region (Fig. 6a). On the contrary, its hybrid materials showed excellent photocatalytic activities in the visible light region (Fig. 6b). We deduce that the enhancement of the photocatalytic activity originates from the synergy between PPy and ZnP2Mo5NR. To illustrate this point clearly, PPy and PPy/ZnP2Mo5NR(M) (the mechanically blended product of PPy and ZnP2Mo5NR) were used as the reference to evaluate the photocatalytic efficiency (Fig. 6c and d). It is notable that their photocatalytic efficiencies are considerably less compared to PPy/ZnP2Mo5NR hybrid materials. This indicates that the synergy between PPy and ZnP2Mo5NR plays a crucial role in the improvement of photocatalytic activity.
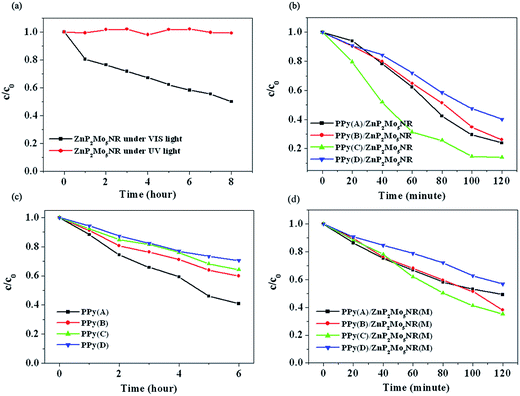 |
| Fig. 6 Degradation rate as a function of time: (a) by ZnP2Mo5NR; (b) by PPy/ZnP2Mo5NR; (c) by PPy; (d) by PPy/ZnP2Mo5NR(M). | |
Furthermore, we also determined that with the increasing ratio of [Py]
:
[APS], the photocatalytic activities of PPy/ZnP2Mo5NR hybrid materials were not raised monotonously. This can also be attributed to the difference in the conductivity of PPy (Fig. 7a). As the ratio of [Py]
:
[APS] > 1, [APS] is insufficient to completely oxidize Py. This will produce PPy with low molecular weight and poor conductivity, which cannot effectively separate photogenerated electron–hole pairs. By increasing the oxidant, PPy with a high molecular weight and conductivity is obtained, and can more effectively separate photogenerated electron–hole pairs. As the ratio of [Py]
:
[APS] = 1, we obtained PPy(C)/ZnP2Mo5NR, which exhibited the best performance during the photocatalytic decomposition of RhB. If [APS] continuously increases, an excess of APS can destroy the π-conjugated structure of PPy and reduce its conductivity and electron–hole pair separation ability. Hence, in these hybrid materials, PPy(C)/ZnP2Mo5NR exhibits the highest photocatalytic efficiency. This is in accordance with the optical and electrochemical studies.
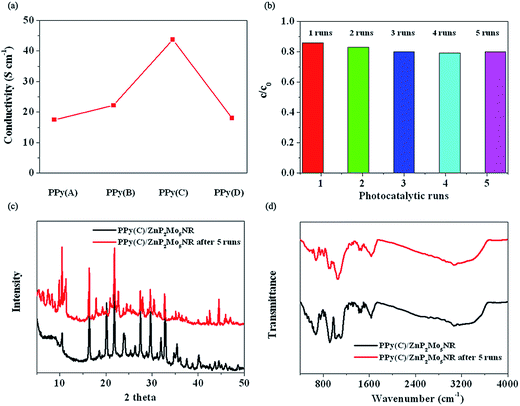 |
| Fig. 7 (a) Conductivities of PPy; (b) cycling runs of the degradation of RhB in the presence of PPy(C)/ZnP2Mo5NR; (c) PXRD of recycled PPy(C)/ZnP2Mo5NR; (d) FTIR of recycled PPy(C)/ZnP2Mo5NR. | |
In the photocatalytic degradation reaction, the activity and stability of the recycled catalyst are very important factors to determine the performance of the photocatalyst. Herein, the photocatalytic properties of PPy(C)/ZnP2Mo5NR were retested five times, and the recycled photocatalysts stilled show excellent photocatalytic properties (Fig. 7b). Furthermore, the recycled sample also exhibited similar PXRD and FTIR patterns to the original hybrid material, which indicates that the structure of PPy(C)/ZnP2Mo5NR is not destroyed during the photocatalysis process (Fig. 7c and d).
Mechanism study
In order to study the intrinsic electronic properties of the PPy/ZnP2Mo5NR hybrid material, a Mott–Schottky measurement was applied in darkness using an impedance technique. We studied the capacitance measurement in the Mott–Schottky-type plot for ZnP2Mo5NR (Fig. 8a). The positive slope of the obtained C−2/E plot illustrates that ZnP2Mo5NR belongs to a typical n-type semiconductor.23 The conductive band potential can also be obtained from this method, and is −0.4 V (vs. SCE). Combined with the band gap (Eg) estimated from DRS, the valence band (VB) of ZnP2Mo5NR was calculated to be 2.72 V (vs. SCE) according to the formula: EVB = ECB + Eg.
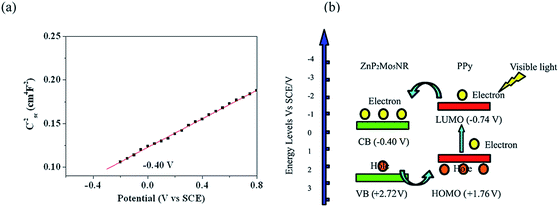 |
| Fig. 8 (a) Mott–Schottky plot of ZnP2Mo5NR; (b) diagram of the photocatalytic mechanism for PPy/ZnP2Mo5NR under visible light. | |
On the basis of these results and our experimental findings, we speculate the mechanism of PPy/ZnP2Mo5NR hybrid material as follows. For PPy/ZnP2Mo5NR, its valence band (VB) and conduction band (CB) match well with the lowest unoccupied molecular orbital (LUMO) and highest occupied molecular orbital (HOMO) of PPy (Fig. 8b). Thus, under visible light irradiation, only PPy is excited and produces an electron from its LUMO orbital, and then the electron transfers into the CB of ZnP2Mo5NR, and at the same time, a hole is formed on VB of ZnP2Mo5NR and injected on to the HOMO of PPy. This process leads to the charge separation and stabilization, which furthermore hinders the recombination of electrons and holes. On the basis of the aforementioned points, in the PPy/ZnP2Mo5NR hybrid material, the role of PPy can be described as an efficient electron donor and good hole transporter.
Conclusions
PPy/CC/POMNR hybrid material was successfully fabricated by the in situ chemical polymerization of Py with CC/POMNR. As expected, compared with CC/POMNR, PPy, and their mechanically blended products, the hybrid material exhibits considerably higher photocatalytic efficiency under the irradiation of visible light. We also discussed the influence of the ratio of [Py]
:
[APS] on the morphology, conductivity and photocatalytic activity of the hybrid material, and an optimal value was obtained. The preparation of PPy/CC/POMNR enables us to establish a rational strategy to improve the photocatalytic performance of POM-based photocatalyst. Furthermore, PPy/CC/POMNR is a new type of efficient photocatalyst for decontaminating colored wastewater produced in textile industries.
Acknowledgements
This work was supported by National Natural Science Foundation of China (21303010, 21273029 and 21471021); Research Foundation for the Doctoral Program of Higher Education of China (20120042110024); Fundamental Research Funds for the Central Universities (N120405005).
References
-
(a) R. Asahi, T. Morikawa, T. Ohwaki, K. Aoki and Y. Taga, Science, 2001, 293, 269 CrossRef CAS PubMed;
(b) W. Zhao, W. H. Ma, C. C. Chen, J. C. Zhao and Z. G. Shuai, J. Am. Chem. Soc., 2004, 126, 4782 CrossRef CAS PubMed;
(c) Y. Cong, J. L. Zhang, F. Chen and M. Anpo, J. Phys. Chem. C, 2007, 111, 6976 CrossRef CAS.
-
(a) S. Kohtani, M. Koshiko, A. Kudo, K. Tokumura, Y. Ishigaki, A. Toriba, K. Hayakawa and R. Nakagaki, Appl. Catal., B, 2003, 46, 573 CrossRef CAS;
(b) S. Kohtani, M. Tomohiro, K. Tokumura and R. Nakagaki, Appl. Catal., B, 2005, 58, 265 CrossRef CAS PubMed;
(c) X. H. Lu, S. L. Xie, H. Yang, Y. X. Tong and H. B. Ji, Chem. Soc. Rev., 2014, 43, 7581–7593 RSC.
-
(a) J. X. Meng, Y. G. Li, H. Fu, X. L. Wang and E. B. Wang, CrystEngComm, 2011, 13, 649 RSC;
(b) K. Wang, D. D. Zhang, J. C. Ma, P. T. Ma, J. Y. Niu and J. P. Wang, CrystEngComm, 2012, 14, 3205 RSC;
(c) W. Q. Kan, J. Yang, Y. Y. Liu and J. F. Ma, Dalton Trans., 2012, 41, 11062 RSC.
-
(a) H. X. Yang, T. F. Liu, M. N. Cao, H. F. Li, S. Y. Gao and R. Cao, Chem. Commun., 2010, 46, 2429 RSC;
(b) Y. H. Guo and C. W. Hu, J. Mol. Catal. A: Chem., 2007, 262, 136 CrossRef CAS PubMed.
-
(a) T. B. Liu, E. Diemann, H. L. Li, A. W. M. Dress and A. Müll, Nature, 2003, 426, 59 CrossRef CAS PubMed;
(b) Z. H. Kang, Y. B. Wang, E. B. Wang, S. Y. Lian, L. Gao, W. S. You, C. W. Hu and L. Xu, Eur. J. Inorg. Chem., 2003, 370 CrossRef CAS;
(c) Z. H. Kang, Y. B. Wang, E. B. Wang, S. Y. Lian, L. Gao, W. S. You, C. W. Hu and L. Xu, Solid State Commun., 2004, 129, 559 CrossRef CAS PubMed.
-
(a) J. X. Meng, Y. Lu, Y. G. Li, H. Fu, X. L. Wang and E. B. Wang, CrystEngComm, 2011, 13, 2479 RSC;
(b) Y. Ding, J. X. Meng, W. L. Chen and E. B. Wang, CrystEngComm, 2011, 13, 2687 RSC;
(c) X. L. Wang, Y. F. Wang, G. C. Liu, A. X. Tian, J. W. Zhang and H. Y. Lin, Dalton Trans., 2011, 40, 9299 RSC.
-
(a) J. Y. Niu, S. W. Zhang, H. N. Chen, J. W. Zhao, P. T. Ma and J. P. Wang, Cryst. Growth Des., 2011, 11, 3769 CrossRef CAS;
(b) B. F. Meng, W. S. You, X. F. Sun, F. Zhang and M. Y. Liu, Inorg. Chem. Commun., 2011, 14, 35 CrossRef CAS PubMed;
(c) Y. Q. Jiao, C. Qin, C. Y. Sun, K. Z. Shao, P. J. Liu, P. Huang, K. Zhou and Z. M. Su, Inorg. Chem. Commun., 2012, 20, 273 CrossRef CAS PubMed.
-
(a) K. Murakoshi, R. Kogure, Y. Wada and S. Yanagida, Sol. Energy Mater. Sol. Cells, 1998, 55, 113 CrossRef CAS;
(b) R. Cervini, Y. Cheng and G. Simon, J. Phys. D: Appl. Phys., 2004, 37, 13 CrossRef CAS.
-
(a) T. Kitamura, M. Maitani, M. Matsuda, Y. Wada and S. Yanagida, Chem. Lett., 2001, 10, 1054 CrossRef;
(b) D. Chowdhury, A. Paul and A. Chattopadhyay, Langmuir, 2005, 21, 4123 CrossRef CAS PubMed.
-
(a) Q. L. Li, C. R. Zhang and J. Q. Li, J. Alloys Compd., 2011, 509, 1953 CrossRef CAS PubMed;
(b) D. Wang, Y. Wang, X. Li, Q. Luo, J. An and H. Yue, Catal. Commun., 2008, 9, 1162 CrossRef CAS PubMed.
-
(a) B. Wang, C. Li, J. F. Pang, X. T. Qing, J. P. Zhai and Q. Li, Appl. Surf. Sci., 2012, 258, 9989 CrossRef CAS PubMed;
(b) S. N. Gu, B. Li, C. J. Zhao, Y. L. Xu, X. Z. Qian and G. R. Chen, J. Alloys Compd., 2011, 509, 5677 CrossRef CAS PubMed;
(c) X. C. Li, G. L. Jiang, G. H. He, W. J. Zheng, Y. Tan and W. Xiao, Chem. Eng. J., 2014, 236, 480 CrossRef CAS PubMed.
-
(a) T. A. Kandiel, R. Dillert and D. W. Bahnemann, Photochem. Photobiol. Sci., 2009, 8, 683 RSC;
(b) F. Deng, L. J. Min, X. B. Luo, S. L. Wu and S. L. Luo, Nanoscale, 2013, 5, 8703 RSC;
(c) N. M. Dimitrijevic, S. Tepavcevic, Y. Z. Liu, T. Rajh, S. C. Silver and D. M. Tiede, J. Phys. Chem. C, 2013, 117, 15540 CrossRef CAS.
-
(a) G. K. R. Senadeera, T. Kitamura, Y. Wada and S. Yanagida, J. Photochem. Photobiol., A, 2006, 184, 234 CrossRef CAS PubMed;
(b) G. K. R. Senadeera, T. Kitamura, Y. Wada and S. Yanagida, J. Photochem. Photobiol., A, 2004, 164, 61 CrossRef CAS PubMed;
(c) X. F. Lu, Q. D. Zhao, X. C. Liu, D. J. Wang, W. J. Zhang, C. Wang and Y. Wei, Macromol. Rapid Commun., 2006, 27, 430 CrossRef CAS.
-
(a) H. Yuvaraj, M. H. Woo, E. J. Park, Y. T. Jeong and K. T. Lim, Eur. Polym. J., 2008, 44, 637 CrossRef CAS PubMed;
(b) Q. Cheng, Y. He, V. Pavlinek, C. Li and P. Saha, Synth. Met., 2008, 158, 953 CrossRef CAS PubMed;
(c) C. Xu, J. Sun and L. Gao, J. Mater. Chem., 2011, 21, 11253 RSC.
-
(a) S. C. Wuang, K. G. Neoh, E. T. Kang, D. W. Pack and D. E. Leckband, J. Mater. Chem., 2007, 17, 3354 RSC;
(b) Z. Zhang, Q. Li, L. Yu, Z. Cui, L. Zhang and G. A. Bowmaker, Macromolecules, 2011, 44, 4610 CrossRef CAS;
(c) F. Deng and Y. X. Li, Colloids Surf., A, 2012, 395, 183 CrossRef CAS PubMed.
-
(a) H. C. Kang and K. E. Gecheler, Polymer, 2000, 41, 6931 CrossRef CAS;
(b) J. Liu and M. X. Wan, J. Mater. Chem., 2001, 11, 404 RSC;
(c) S. Machida and S. Miyata, Synth. Met., 1989, 31, 311 CrossRef CAS;
(d) J. Y. Ouyang and Y. F. Li, Polymer, 1997, 38, 1971 CrossRef CAS.
-
(a)
G. M. Sheldrick, SHELX-97, Program for Crystal Structure Refinement, University of Göttingen, Germany, 1997 Search PubMed;
(b)
G. M. Sheldrick, SHELX-97, Program for Crystal structure Solution, University of Göttingen, Germany, 1997 Search PubMed.
-
(a) X. Wang, J. Peng, D. D. Wang, M. G. Liu, C. L. Meng, A. X. Tian, K. Alimaje and Z. Y. Shi, Inorg. Chim. Acta, 2012, 392, 160 CrossRef CAS PubMed;
(b) Y. Lu, J. Lv, E. B. Wang, Y. Q. Guo, X. X. Xu and L. Xu, J. Mol. Struct., 2005, 740, 159 CrossRef CAS PubMed.
-
(a) X. F. Wang, Y. H. Shen, A. J. Xie, L. G. Qiu, S. Li and Y. Wang, J. Mater. Chem., 2011, 21, 9641 RSC;
(b) H. Zhang, R. L. Zong and Y. F. Zhu, J. Phys. Chem. C, 2009, 113, 4605 CrossRef CAS.
-
(a) C. X. Zhang, Y. G. Chen, Q. Tang, Z. C. Zhang, D. D. Liu and H. X. Meng, Inorg. Chem. Commun., 2012, 17, 155 CrossRef CAS PubMed;
(b) Y. Lu, Y. G. Li, E. B. Wang, J. Lv, L. Xu and R. Clérac, Eur. J. Inorg. Chem., 2005, 1239 CrossRef CAS.
-
(a) S. X. Min, F. Wang and Y. Q. Han, J. Mater. Sci., 2007, 42, 9966 CrossRef CAS;
(b) J. H. Wei, Q. Zhang, Y. Liu, R. Xiong, C. X. Pang and J. Shi, J. Nanopart. Res., 2011, 15, 3157 CrossRef;
(c) S. De, A. Dey and S. K. De, J. Phys. Chem. Solids, 2007, 68, 66 CrossRef CAS PubMed;
(d) L. X. Zhang, P. Liu and Z. X. Su, Polym. Degrad. Stab., 2006, 91, 2213 CrossRef CAS PubMed.
-
(a) H. Liu, S. A. Cheng, M. Wu, H. J. Wu, J. Q. Zhang, W. Z. Li and C. N. Cao, J. Phys. Chem. A, 2000, 104, 7016 CrossRef CAS;
(b) W. H. Leng, Z. Zhang, J. Q. Zhang and C. N. Cao, J. Phys. Chem. B, 2005, 109, 15008 CrossRef CAS PubMed.
-
(a) L. H. Ai, C. H. Zhang, L. L. Li and J. Jiang, Appl. Catal., B, 2014, 148–149, 191 CrossRef CAS PubMed;
(b) L. J. Shen, S. J. Liang, W. M. Wu, R. W. Liang and L. Wu, Dalton Trans., 2013, 42, 13649 RSC;
(c) X. H. Lu, G. M. Wang, S. L. Xie, J. Y. Shi, W. Li, Y. X. Tong and Y. Li, Chem. Commun., 2012, 48, 7717 RSC;
(d) S. L. Xie, T. Zhai, W. Li, M. H. Yu, C. L. Liang, J. Y. Gan, X. H. Lu and Y. X. Tong, Green Chem., 2013, 15, 2434 RSC.
Footnote |
† Electronic supplementary information (ESI) available: Crystal data and structure refinement results, selected bond lengths and angles of ZnP2Mo5. Absorption spectra of RhB degraded with ZnP2Mo5NR under irradiation of ultraviolet and visible light; absorption spectra of RhB degraded with PPy/ZnP2Mo5NR, PPy and PPy/ZnP2Mo5NR(M) under irradiation of visible light. CCDC 1017803. For ESI and crystallographic data in CIF or other electronic format see DOI: 10.1039/c4ta05071a |
|
This journal is © The Royal Society of Chemistry 2015 |
Click here to see how this site uses Cookies. View our privacy policy here.