A recyclable carbon nanoparticle-based fluorescent probe for highly selective and sensitive detection of mercapto biomolecules†
Received
14th August 2014
, Accepted 22nd October 2014
First published on 27th October 2014
Abstract
Carbon nanoparticles (CNPs) with strong blue emission are synthesized using a microwave-assisted hydrothermal method. The fluorescence of the CNPs can be completely quenched by Hg2+ through an effective electron or energy transfer process due to the synergetic strong electrostatic interaction and metal–ligand coordination. Based on this, a system containing Hg2+-quenched CNPs (CNP-Hg2+) is designed to be a sensitive and selective turn-on fluorescent probe towards cysteine (a type of mercapto biomolecule) with a detection limit of 15 nM. The fluorescence of CNP-Hg2+ aqueous solution can be repeatedly turned on and off for over 10 times by alternative addition of cysteine and Hg2+, respectively. After 10 cycles, the fluorescence intensity could be recovered to as high as 85% of the original value of CNPs. Remarkably, the sensing process is able to be observed by the naked eye under UV irradiation. Furthermore, the sensing is specific to biothiols and the sensor is able to work in living cells.
Introduction
Mercapto biomolecules including cysteine (Cys), homocysteine (Hcy), and glutathione (GSH) play a crucial role in cellular antioxidant defense systems and in maintaining biological redox homeostasis through the equilibrium established between reduced free thiols (RSH) and oxidized disulfides (RSSR).1–3 An abnormal level of thiols in the biological system can be regarded as an indicator of health issues such as liver damage, skin lesions, cardiovascular diseases, and cancers.4–7 The specific and sensitive determination of mercapto biomolecules is thus of particular interest in biological and toxicological diagnosis.
Conventional analytical assays like mass spectrometry, gas chromatography, high-performance liquid chromatography and electrochemical methods generally require expensive instruments and/or sophisticated sample preparation. These issues stimulate the development of new techniques for sensing thiols.8–11
Recently, fluorescent sensors for detecting biomolecules have attracted increasing attention due to their superiority in virtue of their high sensitivity, simplicity, rapid response, and the capability to be applied in both solution and solid phases.12–16 Various organic dyes and semiconductor quantum dot probes have been reported for detecting mercapto biomolecles.17–20 However, their problems on water solubility, photostability, and/or pH-stability still need to be resolved for practical applications. Moreover, few organic dye-based sensors display fluorescence reversibility which is essential for real-time monitoring of the dynamic biological processes in living cells.21,22 To achieve a desirable technology for sensing mercapto biomolecules with combined superior advantages, a variety of materials is currently being actively developed. Among the efforts, the recent discovery of the photoluminescence effect of carbon nanoparticles (CNPs) has attracted increasing research interest.23 Compared to traditional semiconductor quantum dots and organic polymer dots, these carbon nanomaterials are featured with a number of strengths covering outstanding chemical inertness, excellent photo-stability, favourable biocompatibility, and good water solubility.24–37 As an excellent fluorescent material, through surface functionalization and/or combination with other nanomaterials, CNPs have been used as probes for sensing various metal ions and biomolecules including proteins.38–44 For example, Shi et al. reported a dual-mode nanosensor with both colorimetric and fluorometric readout based on integration of CNPs and gold nanoparticles for detection of GSH in distilled water.45 However, the fluorescence intensity increased only by ∼25% upon addition of 3 μM of GSH and a long response time of ∼5 min was needed. Moreover, the influence of pH values on the sensing characteristics and the reversibility of the probes were not presented. Table S1† summarizes the sensing performance of some recently reported fluorescence thiol sensors based on CNPs. It can be noted that the response time, pH stability, PL recovering efficiency, and recyclability need to be further improved for practical applications.
In this work, water-soluble CNPs with a fluorescence quantum yield of about 0.3 are prepared by a simple microwave-assisted hydrothermal method using melamine and trisodium citrate dihydrate as precursors. As shown in Scheme 1, we purposely quench the strong blue emission of CNPs and use the integrated system of Hg2+-quenched CNPs (CNP-Hg2+) as a non-fluorescent sensor. This sensor can be conveniently employed as a fluorescence turn-on sensor for sensitive detection of mercapto biomolecules such as Cys, Hcy and GSH. Attractively, the sensor is able to be regenerated by further addition of Hg2+ and reused for over 10 times. Furthermore, such a CNP-Hg2+ system is capable of imaging mercapto biomolecules in living cells.
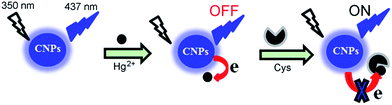 |
| Scheme 1 Schematic diagram showing the fabrication process of the CNP-Hg2+ sensor. The fluorescence of the sensor can be turned on by addition of Cys. | |
Experimental
Materials
Melamine, trisodium citrate dihydrate, N-ethylmaleimide (NEM), NaCl, KCl, Mn(OAc)2·4H2O, Co(OAc)2, Ni(OAc)2, Al(NO3)3·9H2O, Cu(OAc)2·H2O, FeCl3, Cs(OAc)2·H2O, CeCl3·7H2O, LiNO3, Ba(OAc)2, MgCl2·6H2O, CdSO4, HgCl2, HEPES, RNA, DNA, cysteine (Cys), homocysteine (Hcy), glutathione (GSH), serine (Ser), valine (Val), tyrosine (Tyr), leucine (Leu), tryptophan (Trp), alanine (Ala), aspartic acid (Asp), methionine (Met), threonine (Thr), isoleucine (Ile), glycine (Gly), argine (Arg), lysine (Lys), and 3-(4,5-dimethylthiazol-2-yl)-2,5-diphenyltetrazolium bromide (MTT) were purchased from Sigma-Aldrich Co. LLC. All chemicals were used as received without further purification. Deionized water with a conductivity of 18.2 MΩ cm−1 was purified through a Millipore water purification system.
Characterizations
The absorption spectra were recorded on a Shimadzu 1700 spectrophotometer using 10 mm path length quartz cuvettes in the range of 250–600 nm, while the fluorescence measurements were carried out on a Horiba Fluormax-4 spectrophotometer at room temperature. X-ray photoelectron spectroscopy (XPS) analysis was performed on a VG ESCALAB 220i-XL surface analysis system. The X-ray diffraction (XRD) pattern was obtained using an X-ray diffractometer (Bruker, D2 PHASER). Fourier transform infrared spectroscopy (FTIR) was performed on an IFS 66 V/S (Bruker) IR spectrometer in the range of 400–4000 cm−1. Transmission electron microscopy (TEM) was performed on a Philips CM200 electron microscope. Fluorescence quantum yield was determined according to an established procedure with quinine sulfate in 0.1 M H2SO4 as a standard (54%). All pHs were measured with a Eutech pH-meter PH 700.
Preparation of fluorescent CNPs
CNPs were prepared by microwave-assisted hydrothermal treatment of melamine and trisodium citrate dihydrate. In a typical synthesis, 0.12 g of melamine and 0.58 g of trisodium citrate dihydrate were added into 25 mL of H2O. Then the mixture solution was transferred into a microwave reactor and maintained at 180 °C for 12 hours. After cooling to room temperature, the CNPs were collected by removing the large particles through filtering using 0.22 μm membranes, and dialyzed using distilled water for two days. CNP aqueous solution was stored at 4 °C for future characterization and use.
Sensor preparation and its application for sensing Cys
10 μM of Hg2+ was added to 4 μg mL−1 of the as-synthesised CNPs to make a sensor (CNP-Hg2+). This integrated sensor does not possess fluorescence and was used for sensing Cys. In a typical run, 40 μL of CNP dispersion solution (0.2 mg mL−1) was added into 2 mL HEPES buffer solution (10 mM, pH 7.2), and then 10 μL of Hg2+ (2 mM) was added to the CNP solution, followed by the addition of a calculated amount of Cys. The sensitivity and selectivity measurements were conducted in triplicate. After the test, the sensor was regenerated by adding 10 μM of Hg2+ ions.
Cell culture and in vitro imaging studies
A549 cells were obtained from the Peking Union Medical College and cultured in culture media (DMEM/F12 supplemented with 10% FBS, 50 unit mL−1 penicillin, and 50 μg mL−1 of streptomycin) at 37 °C in a humidified incubator containing 5% CO2. For cell imaging studies, cells were seeded in a 6-well plate at a density of 104 cells per well in culture media and maintained at 37 °C in a 5% CO2/95% air incubator for 24 hours. A549 cells were pretreated with 4 mM NEM for 1 hour to reduce the concentration of thiol-containing biomolecules, and then they were incubated with 100 μL of CNP aqueous solution in culture media for another 4 hours at 37 °C. After the medium was removed and the cells were carefully washed with PBS twice, the cells were then incubated with 20 μM Hg2+ aqueous solution for another 5 minutes at 37 °C. Fluorescence imaging of living A549 cells was performed under a Nikon fluorescence microscope (excitation light source: 330–380 nm). Lastly, the cells treated with NEM, CNPs and Hg2+ were incubated with 50 μM Cys aqueous solution for another 5 minutes at 37 °C, and imaged using fluorescence microscopy. Confocal laser scanning microscopy images of A549 cells incubated with CNPs were obtained using a Leica SPE confocal laser scanning microscope with a 405 nm laser.
MTT assay
A549 cells were seeded in a 6-well plate at a density of 104 cells per well in culture media and maintained at 37 °C in a 5% CO2/95% air incubator for 24 hours. Then, the culture media were removed and the cells were incubated in culture medium containing the as-prepared CNPs with different concentrations (0–500 μg mL−1) for 24 hours in the presence of 10 μM Hg2+ and washed with the culture medium. An amount of 200 μL of the new culture medium (without FBS) containing MTT (20 μL, 5 mg mL−1) was then added, followed by incubation for 4 hours to allow the formation of formazan crystals. Absorbance was measured at 570 nm. Cell viability values were determined according to the following formula: cell viability (%) = the absorbance of the experimental group/the absorbance of the control group × 100%.
Results and discussion
Structure and composition of CNPs
Fig. 1a shows a TEM image of CNPs. The inset histogram reveals a size distribution of nanoparticles around 19.5 nm. High-resolution TEM (HRTEM) observation in Fig. 1b further shows that a nanoparticle is composed of several crystalline carbon quantum dots (left inset in Fig. 1b) embedded in an amorphous matrix. The right inset depicts a diameter distribution of quantum dots around 2.6 nm. Fig. 1c is a typical XRD pattern which presents only one broader peak at 2θ = 24°. This is consistent with the result shown in previous reports of CNPs.46,47 The Fourier transform infrared (FTIR) spectrum was acquired to determine the surface functional groups of the CNPs and the result is in Fig. 1d. The peak at 3449 cm−1 is ascribed to the characteristic absorption band of N–H stretching vibration mode and the peaks at 3256 and 1106 cm−1 are attributed to the stretching vibration of –OH. The peaks at 1600 and 1400 cm−1 indicate the existence of COO−. The characteristic absorption band of C–OH stretching vibration at 1289 cm−1 is also observed, and the peaks at 2923 and 815 cm−1 can be assigned to the C–H stretching vibration mode and C–H out-of-plane bending mode.
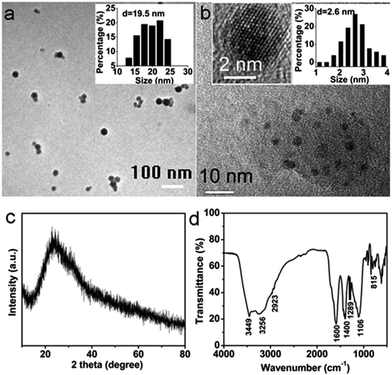 |
| Fig. 1 (a) and (b) TEM images of CNPs. The insets show the particle size distribution histograms (n = 50) and HRTEM image of CNPs. (c) The XRD pattern and (d) FTIR spectrum of the CNPs. | |
The surface composition and elemental analysis for the CNPs were further characterized by X-ray photoelectron spectroscopy (XPS). Three peaks at about 284.5, 399.5 and 531.5 eV are presented in the XPS survey spectrum of CNPs (Fig. 2a) and correspond to C1s, N1s and O1s core levels, respectively. The C/N/O atom ratio was calculated to be 57.0/3.9/39.1. The high-resolution XPS spectrum of C1s (Fig. 2b) can be resolved into three peaks with binding energies of about 284.2, 285.0 and 287.9 eV, corresponding to C–C, C–N and C
N/C
O, respectively. Deconvolution of the N1s spectrum in Fig. 2c shows three peaks at 398.5, 399.6 and 400.4 eV for C–N–C, N–(C)3 and N–H bonds, respectively. The fitting of the O1s peak gives three components at 531.0, 532.2 and 535.3 eV for C
O, C–OH/C–O–C and COOH groups, respectively (Fig. 2d). The XPS analysis agrees well with the above FTIR observations. These findings suggest that the surfaces of the CNPs were functionalized with amino, hydroxyl and carboxylic/carbonyl moieties originated from melamine and trisodium citrate dihydrate.
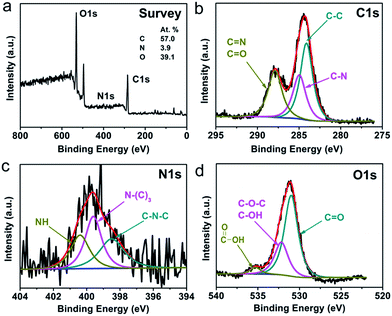 |
| Fig. 2 (a) XPS survey spectrum, and (b) C1s, (c) N1s and (d) O1s high-resolution spectra of the CNPs. | |
The zeta-potential of the CNP aqueous solution was measured to be −56.7 mV (Fig. S1†).
Fig. 3a presents the normalized UV-vis absorption and PL spectra of CNPs dispersed in aqueous solution. It reveals that CNPs have an absorption peaked at 342 nm and a blue emission peaked at 437 nm when excited at 350 nm. No obvious wavelength shift is observed in the PL spectra of CNPs as the excitation wavelength varies (Fig. S2†). In line with the PL measurement, the CNP aqueous solution under UV light (365 nm) exhibits a strong blue fluorescence (Fig. 3a, inset) with a fluorescence quantum yield of 0.3 using quinine sulfate as a reference.48–50 Attractively, the PL intensity of these CNPs can be maintained even under high ionic strength conditions (1 mol L−1 NaCl solution, Fig. S3†) or after irradiation for 1 hour by a 350 nm xenon lamp equipped in the FL spectrometer (Fig. S4†). Analysis of the fluorescence decay kinetics at 437 nm revealed a single exponential decay with a lifetime of 7.0 ns (Fig. S5†), suggesting the singlet state nature of the emission.
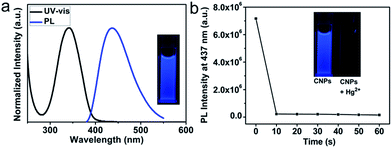 |
| Fig. 3 (a) Normalized UV-vis absorption (black line) and PL (blue line) spectra of the CNPs in aqueous solution (λex = 350 nm). Inset: the photograph of CNP solution under UV light (365 nm). (b) The variation of PL intensity of CNPs at 437 nm upon addition of 10 μM of Hg2+ in HEPES buffer solution; the observation time interval is 10 s. The inset shows the photograph of CNP solutions in the absence and presence of 10 μM Hg2+ under UV light. | |
CNP-Hg2+ for sensing biothiols as a fluorescence turn-on sensor
The strong blue emission of CNP solution can be completely quenched in 10 seconds upon addition of 10 μM Hg2+ solution, as shown in Fig. 3b and S6.† The quenching is able to be directly observed by the naked eye under UV light (Fig. 3b, inset). Prolonging the reaction time does not lead to a further reduction of PL intensity. The fluorescence quenching of CNPs by Hg2+ has been elucidated to be presumably due to nonradiative electron/hole annihilation through an effective electron or energy transfer process resulting from the strong electrostatic interaction and metal–ligand coordination between CNPs and Hg2+.38,44
In this non-fluorescent CNP-Hg2+ system, if we add mercapto biomolecules including Cys, Hcy, and GSH, the fluorescence can be dramatically increased due to a previously described competition mechanism.51,52 The time-dependent fluorescence spectra of the CNP-Hg2+ against 10 μM Cys in HEPES buffer solution (10 mM, pH 7.2) was collected at room temperature. The spectra are shown in Fig. S7† and the fluorescence intensities at different times are shown in Fig. 4a. Obviously, the PL intensity of CNP-Hg2+ aqueous solution dramatically increases within 10 seconds upon addition of 10 μM Cys. These results verified that the CNP-Hg2+ system can function as a fluorescence turn-on sensor with a fast response for biothiols. The thiol groups as strong Hg2+ chelators are able to remove Hg2+ from CNP surfaces through the formation of Hg–S bonds.
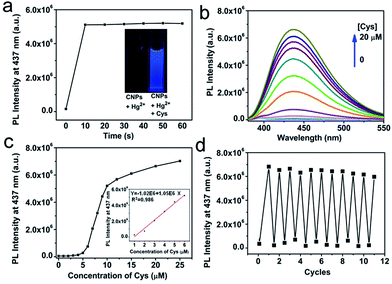 |
| Fig. 4 (a) The variation of PL intensity of CNP-Hg2+ at 437 nm upon addition of 10 μM of Cys in HEPES buffer solution; the observation time interval is 10 s. The inset shows the photograph of the solution in the absence and presence of Cys under UV light. (b) Dependence of PL spectra of CNP-Hg2+ in HEPES buffer solution (10 mM, pH 7.2) on the gradual addition of Cys from 0 to 20 μM. (λex = 350 nm). (c) Dependence of PL intensity at 437 nm with incremental addition of Cys. Inset: PL intensity of CNP-Hg2+ solution at 437 nm versus the concentrations of Cys, the measurements were performed using CNP-Hg2+ solution with 4 μM Cys pre-added as the. (d) The PL intensity at 437 nm of CNP-Hg2+ in HEPES buffer solution upon alternative addition of 20 μM of Cys and 10 μM of Hg2+. The concentrations of Hg2+ and Cys in the last cycle were 0.5 mM and 1.0 mM, respectively. | |
The effects of the synthetic parameters of CNPs including the molar ratio of precursors, reaction time and reaction temperature on their sensing properties were studied, as summarized in Table S2.† It can be seen that the CNPs prepared under different conditions show similar UV-vis spectra (Fig. S8†), and their PL emission peaks have no obvious shift under excitation for wavelengths varying from 300 nm to 400 nm. A maximum PL intensity at about 437 nm was observed for all samples under the excitation at 350 nm. Furthermore, the fluorescence quantum yields (QYs) of all CNPs were calculated to be around 0.3 with excitation at different wavelengths. For all samples, their PL emissions could be quenched by Hg2+ and then recovered by Cys, as shown in Fig. S9(A–G).† However, the PL quenching efficiency and recovering efficiency were different for the samples prepared under different reaction conditions. The CNPs prepared using the conditions described in the Experimental section (denoted as CNP-D in Table S2†) showed the best overall performance and were therefore used as the sensing platform in this work.
Absorption and fluorescence titration experiments were carried out to illustrate the spectral response of our CNP-Hg2+ system to Cys of different concentrations. As shown in Fig. S10a,† the absorption peak of CNP-Hg2+ at 318 nm red-shifts to 335 nm in the presence of Cys. Considering that there is no obvious absorbance of Hg2+, Cys and Hg2+-Cys solutions in the wavelength range of from 300 to 400 nm (Fig. S10b†), the observed red-shift should be attributed to the interactions between Cys with Hg2+ and groups (such as amino, hydroxyl and carboxylic/carbonyl) on the CNP surfaces. The dependence of the PL of CNP-Hg2+ on the concentration of Cys is shown in Fig. 4b and c. The increase of the concentration of Cys from 0 to 4 μM only slightly changes the PL intensity. However, further addition of Cys leads to a sharp increase of PL intensity. Based on these results, it is ideal to prepare a CNP-Hg2+ solution containing 4 μM Cys for sensor applications. To demonstrate this, the dependence of PL intensity of the CNP-Hg2+ solution with 4 μM Cys at 437 nm on the concentrations of further added Cys is presented in the inset of Fig. 4c. A good linear relationship between the added Cys and fluorescence intensity is obtained; and the limit of detection, defined as three times of the standard deviation of background,53,54 was calculated to be 15 nM. These results indicate that CNP-Hg2+ system can be applied as a fluorescence turn-on sensor for Cys detection in aqueous solutions with a reasonably high sensitivity.55–57
Remarkably, the sequential addition of constant amounts of Cys and Hg2+ to the aqueous solution of CNP-Hg2+ gives rise to an alternative change of the system's PL intensity, as illustrated in Fig. 4d. These reversible fluorescence “ON-OFF” processes could be repeated for more than 10 times with the “ON”-state fluorescence intensity retention of over 85% of the initial fluorescence of CNPs. Even in the presence of 0.5 mM Hg2+, the PL of CNPs is able to be recovered through addition of 1 mM Cys.
The specificity of CNP-Hg2+ sensor to mercapto thiol molecules
The complexity of intracellular systems presents great challenges to mercapto biomolecule detection. These are not only in the aspect of sensitivity but more importantly in the aspect of selectivity because there are many types of amino acids, DNA and RNA in living systems. To verify the selectivity of the CNP-Hg2+ system to mercapto biomolecules, the PL response of CNP-Hg2+ solution to other amino acids, RNA, and DNA was monitored and the results are presented in Fig. 5a. It is clear that only thiol-containing compounds (Cys, Hcy and GSH) result in a significant PL enhancement (Fig. S11†). In contrast, other amino acids such as Ser, Val, Tyr, Leu, Trp, Ala, Asp, Met, Thr, Ile, Gly, Arg, and Lys as well as RNA and DNA do not induce any obvious changes of the system's PL properties (Fig. 5b, black columns). Notably, even in the presence of these species, the PL of CNP-Hg2+ solution can be recovered by addition of Cys (Fig. 5a, red columns). These results clearly suggested that the CNP-Hg2+ system has an excellent selectivity to biothiols. However, our experiments also revealed that, S2− could also completely recover the PL of the CNP-Hg2+ solution due to its high affinity with Hg2+. Moreover, addition of histidine increased the PL of CNP-Hg2+ solution slightly, but did not affect the PL recovery. In contrast, excessive Ag+ had negligible influence on the PL of CNP-Hg2+ solution but caused a reduced PL recovery efficiency, as illustrated in Fig. S12.†
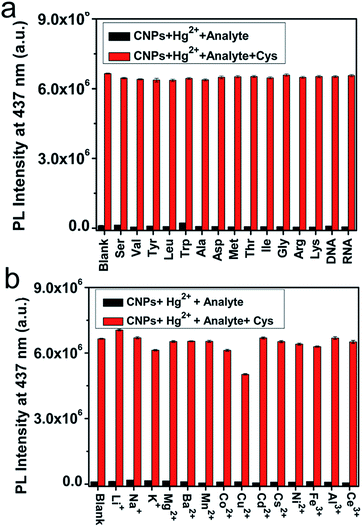 |
| Fig. 5 (a) Selectivity of the CNP-Hg2+ system to Cys in the presence of amino acids or DNA, RNA in HEPES buffer solution (10 mM, pH 7.2). (b) Influence of impurity metal ions on the performance of the CNP-Hg2+ sensor. The black and red columns refer to the CNP-Hg2+ mixture solution in the absence and presence of 20 μM Cys, respectively. Blank refers to free CNP-Hg2+ mixture solution. The error bars represent standard deviations based on three independent measurements. The concentration of competing species was 20 μM. | |
Influence of impurity metal ions on the performance of the CNP-Hg2+ sensor
It is important for a sensor to work in the interference of other parameters. As one test case, we studied the interference of other metal ions, including Li+, Na+, K+, Mg2+, Ba2+, Mn2+, Co2+, Cu2+, Cd2+, Cs2+, Ni2+, Fe3+, Al3+, and Ce3+, to the sensing performance of CNP-Hg2+ in HEPES buffer solution (10 mM, pH 7.2). As illustrated in Fig. 5b (black columns), the PL intensities at 437 nm are relatively constant in the presence of all of the tested metal ions, probably due to the stronger affinity of Hg2+ with the amino and carboxylic groups on CNP surfaces as compared with the added metal ions. Furthermore, it is also observed that the PL of CNP-Hg2+ solution can be greatly increased upon addition of Cys even in the presence of these interfering metal ions (Fig. 5b, red columns).
Influence of pH on the performance of the CNP-Hg2+ sensor
The effect of pH values on the PL stability of the CNP-Hg2+ system was also investigated. Fig. 6 shows the pH-dependent PL intensities at 437 nm of CNP-Hg2+, and CNP-Hg2+/Cys in aqueous solutions. It is found that the PL of CNP-Hg2+ is constantly low within the pH range from 5 to 9 (red line) and, upon addition of Cys, the PL of the CNP-Hg2+ system massively enhances and maintains a relatively stable value (green line). These findings demonstrate that the CNP-Hg2+ system is very stable in the pH range of 5 to 9 which covers physiological conditions. Hence, the excellent pH stability of the CNP-Hg2+ system suggests its great potential for intercellular sensing and imaging.
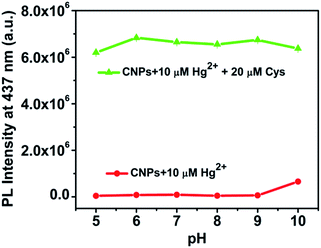 |
| Fig. 6 Effect of pH on the PL intensity at 437 nm of CNP-Hg2+ (red line) and CNP-Hg2+/Cys (green line) solutions at room temperature. | |
In a recent study by Zhou et al.,44 CNPs were prepared by pyrolysis of ethylenediamine-tetraacetic acid (EDTA) salts at 400 °C under flowing N2. It was observed that the fluorescence quantum yield of CNPs decreases from 11.0% to 8.9% at 5 minutes after addition of Hg2+, and the fluorescence intensity at 410 nm increases by ∼30% upon addition of 2 eq. Cys at pH 8.5. The relatively low quenching efficiency and its strong pH dependence may be due to the presence of only some hydroxyl and carboxylic surface groups on the CNPs synthesized at high temperature. In comparison, our CNPs are prepared under moderate conditions, and their surfaces are rich with amino, hydroxyl and carboxylic/carbonyl moiety functional groups. These groups, in particular the amino group, serve as a key acceptor to Hg2+, and thus the PL of our CNPs is completely quenched by Hg2+ and recovered by Cys in a wide pH range, enabling the CNP-Hg2+ system to be an effective sensor for sensing mercapto biomolecules with high sensitivity and selectivity.
Detection and bioimaging of Cys in living cells
Once we demonstrate that the CNP-Hg2+ system has high sensitivity and specificity for the detection of mercapto thiols and can work with high performance at different pHs and along with the interference of various metal ions, the next step is to test whether our approach can be used for the detection of biothiols in living systems. Before the test, it is essential to measure the cytotoxicity of the CNP-Hg2+ system. This was performed with MTT assays and the results are in Fig. 7a. The viability of the A549 cells does not show an obvious decrease in comparison to that of the control group (without addition of CNP-Hg2+ ions). Owing to the large amount of mercapto biomolecules in the cell, a concentration of Hg2+ (10 μM) under our experimental conditions does not induce obvious cell death. Furthermore, a z-stack of confocal laser scanning microscopy images verifies that the CNPs can be uptaken into the cells, rather than just being adsorbed on the surfaces of cells (Fig. S13 and S14†).58,59 Thus, the as-prepared CNP-Hg2+ system can be considered to be with low cytotoxicity and biocompatible for the detection of biothiols in living cells.
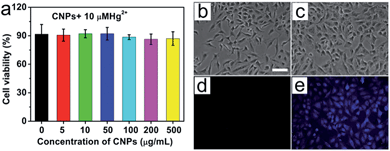 |
| Fig. 7 (a) Cell viability values (%) estimated by MTT proliferation tests versus incubation concentrations of CNPs (0, 5, 10, 50, 100, 200, and 500 μg mL−1) in the presence of 10 μM Hg2+ at 37 °C for 24 h. Bright-field and fluorescence images of A549 cells incubated with CNP-Hg2+ (b and d) and CNP-Hg2+/Cys (c and e). The scale bar is 40 μm. | |
The application of the CNP-Hg2+ system for detecting mercapto biomolecules in living cells was demonstrated. Fig. 7b–e shows the bright field and fluorescence images of living A549 cells incubated with CNP-Hg2+ and CNP-Hg2+/Cys. As the cells were incubated with CNP-Hg2+, no obvious fluorescence signal was observed (Fig. 7d). When these cells were further incubated with foreign addition of Cys, obvious strong blue fluorescence can be observed (Fig. 7e) due to the decomplexation of intracellular CNP-Hg2+ to fluorescence CNPs by Cys uptake, demonstrating the potential of the CNP-Hg2+ system for thiol sensing in living cells.
Conclusions
In summary, water-soluble CNPs with strong blue emission were synthesized, and their fluorescence could be completely quenched by Hg2+. Based on that, a highly selective and sensitive CNP-Hg2+ fluorescence sensor for mercapto biomolecules was designed. The CNP-Hg2+ system exhibits a rapid fluorescence turn on response to Cys with a detection limit of as low as 15 nM, and the sensor can be regenerated for recyclable use by subsequent addition of Hg2+. After 10 recycles of use, the PL intensity of the system can still achieve as high as 85% of the original PL value of CNPs. Notably, This sensor is very specific to mercapto thiols and can work with high performance at different pHs and along with the interference of a variety of metal ions. With these superior advantages, the CNP-Hg2+ system is capable of in vivo detection of biothiols.
Acknowledgements
This work was supported by the Research Grants Council of the Hong Kong Special Administrative Region, China (Project no. CityU 102010) and National Natural Science Foundation of China (NSFC Grants 51372213 and 51172244).
Notes and references
- J. D. Finkelstein and J. J. Martin, Int. J. Biochem. Cell Biol., 2000, 32, 385 CrossRef CAS.
- S. M. Deneke, Curr. Top. Cell. Regul., 2000, 36, 151 CAS.
- X. G. Chen, Y. Zhou, X. J. Peng and J. Yoon, Chem. Soc. Rev., 2010, 39, 2120 RSC.
- L. A. Herzenberg, S. C. De Rosa, J. G. Dubs, M. Roederer, M. T. Anderson, S. W. Ela, S. C. Deresinski and L. A. Herzenberg, Proc. Natl. Acad. Sci. U. S. A., 1997, 94, 1967 CrossRef CAS.
- S. Shahrokhian, Anal. Chem., 2001, 73, 5972 CrossRef CAS.
-
R. Carmel and D. W. Jacobsen, Homocysteine in Health and Disease, Cambridge University Press, Cambridge, U.K, 2001 Search PubMed.
- D. M. Townsend, K. D. Tew and H. Tapiero, Biomed. Pharmacother., 2003, 57, 145 CrossRef CAS.
- P. Capitan, T. Malmezat, D. Breuillé and C. Obled, J. Chromatogr. B: Biomed. Sci. Appl., 1999, 732, 127 CrossRef CAS.
- Y. Hiraku, M. Murata and S. Kawanishi, Biochim. Biophys. Acta, 2002, 1570, 47 CrossRef CAS.
- X. Guan, B. Hoffman, C. Dwivedi and D. P. Matthees, J. Pharm. Biomed. Anal., 2003, 31, 251 CrossRef CAS.
- W. Chen, Y. Zhao, T. Seefeldt and X. M. Guan, J. Pharm. Biomed. Anal., 2008, 48, 1375 CrossRef CAS PubMed.
- C. Lodeiro, J. L. Capelo, J. C. Mejuto, E. Oliveira, H. M. Santos, B. Pedras and C. Nunez, Chem. Soc. Rev., 2010, 39, 2948 RSC.
- J. S. Wu, W. M. Liu, J. C. Ge, H. Y. Zhang and P. F. Wang, Chem. Soc. Rev., 2011, 40, 3483 RSC.
- M. H. Lan, J. S. Wu, W. M. Liu, W. J. Zhang, J. C. Ge, H. Y. Zhang, J. Y. Sun, W. W. Zhao and P. F. Wang, J. Am. Chem. Soc., 2012, 134, 6685 CrossRef CAS PubMed.
- M. H. Lan, W. M. Liu, J. C. Ge, J. S. Wu, H. J. Wang, W. J. Zhang, Y. F. Bi and P. F. Wang, Chem. Commun., 2012, 48, 6818 RSC.
- M. H. Lan, W. M. Liu, Y. Wang, J. C. Ge, J. S. Wu, H. Y. Zhang, J. H. Chen, W. J. Zhang and P. F. Wang, ACS Appl. Mater. Interfaces, 2013, 5, 2283 CAS.
- W. H. Wang, J. O. Escobedo, C. M. Lawrence and R. M. Strongin, J. Am. Chem. Soc., 2004, 126, 3400 CrossRef CAS PubMed.
- H. Maeda, H. Matsuno, M. Ushida, K. Katayama, K. Saeki and N. Itoh, Angew. Chem., Int. Ed., 2005, 44, 2922 CrossRef CAS PubMed.
- W. Y. Lin, L. L. Long, L. Yuan, Z. M. Cao, B. B. Chen and W. Tan, Org. Lett., 2008, 10, 5577 CrossRef CAS PubMed.
- S. Huang, Q. Xiao, R. Li, H. L. Guan, J. Liu, X. R. Liu, Z. K. He and Y. Liu, Anal. Chim. Acta, 2009, 645, 73 CrossRef CAS PubMed.
- J. S. Wu, R. L. Sheng, W. M. Liu, P. F. Wang, J. J. Ma, H. Y. Zhang and X. Q. Zhuang, Inorg. Chem., 2011, 50, 6543 CrossRef CAS PubMed.
- A. Y. Cho and K. H. Choi, Chem. Lett., 2012, 41, 1611 CrossRef CAS.
- X. Y. Xu, R. Ray, Y. L. Gu, H. J. Ploehn, L. Gearheart, K. Raker and W. A. Scrivens, J. Am. Chem. Soc., 2004, 126, 12736 CrossRef CAS PubMed.
- S. N. Baker and G. A. Baker, Angew. Chem., Int. Ed., 2010, 49, 6726 CrossRef CAS PubMed.
- S. N. Qu, X. Y. Wang, Q. P. Lu, X. Y. Liu and L. J. Wang, Angew. Chem., Int. Ed., 2012, 51, 12215 CrossRef CAS PubMed.
- C. Y. Wu, C. Wang, T. Han, X. J. Zhou, S. W. Guo and J. Y. Zhang, Adv. Healthcare Mater., 2013, 2, 1613 CrossRef CAS PubMed.
- R. Q. Ye, C. S. Xiang, J. Lin, Z. W. Peng, K. W. Huang, Z. Yan, N. P. Cook, E. L. G. Samuel, C. C. Hwang, G. D. Ruan, G. Ceriotti, A. R. O. Raji, A. A. Marti and J. M. Tour, Nat. Commun., 2013, 4, 2943 Search PubMed.
- S. Liu, J. Q. Tian, L. Wang, Y. W. Zhang, X. Y. Qin, Y. L. Luo, A. M. Asiri, A. O. Al-Youbi and X. P. Sun, Adv. Mater., 2012, 24, 2037 CrossRef CAS PubMed.
- X. Y. Qin, W. B. Lu, A. M. Asiri, A. O. Al-Youbi and X. P. Sun, Catal. Sci. Technol., 2013, 3, 1027 CAS.
- X. Y. Qin, S. Liu, W. B. Lu, H. Y. Li, G. H. Chang, Y. W. Zhang, J. Q. Tian, Y. L. Luo, A. M. Asiri, A. O. Al-Youbi and X. P. Sun, Catal. Sci. Technol., 2012, 2, 711 CAS.
- S. Liu, J. Q. Tian, L. Wang, Y. L. Luo and X. P. Sun, RSC Adv., 2012, 2, 411 RSC.
- S. Liu, L. Wang, J. Q. Tian, J. F. Zhai, Y. L. Luo, W. B. Lu and X. P. Sun, RSC Adv., 2011, 1, 951 RSC.
- S. Liu, J. Q. Tian, L. Wang, Y. L. Luo, J. F. Zhai and X. P. Sun, J. Mater. Chem., 2011, 21, 11726 RSC.
- X. Y. Qin, W. B. Lu, A. M. Asiri, A. O. Al-Youbi and X. P. Sun, Sens. Actuators, B, 2013, 184, 156 CrossRef CAS PubMed.
- X. Y. Qin, A. M. Asiri, K. A. Alamry, A. O. Al-Youbi and X. P. Sun, Electrochim. Acta, 2013, 95, 260 CrossRef CAS PubMed.
- W. B. Lu, X. Y. Qin, A. M. Asiri, A. O. Al-Youbi and X. P. Sun, J. Nanopart. Res., 2013, 15, 1344 CrossRef.
- X. Y. Qin, W. B. Lu, G. H. Chang, Y. L. Luo, A. M. Asiri, A. O. Al-Youbi and X. P. Sun, Gold Bull., 2012, 45, 61 CrossRef CAS PubMed.
- W. B. Lu, X. Y. Qin, S. Liu, G. H. Chang, Y. W. Zhang, Y. L. Luo, A. M. Asiri, A. O. Al-Youbi and X. P. Sun, Anal. Chem., 2012, 84, 5351 CrossRef CAS PubMed.
- A. W. Zhu, Q. Qu, X. L. Shao, B. Kong and Y. Tian, Angew. Chem., Int. Ed., 2012, 51, 7185 CrossRef CAS PubMed.
- Y. H. Wang, L. Bao, Z. H. Liu and D. W. Pang, Anal. Chem., 2011, 83, 8130 CrossRef CAS PubMed.
- S. Maiti, K. Das and P. K. Das, Chem. Commun., 2013, 49, 8851 RSC.
- X. Y. Ouyang, J. H. Liu, J. S. Li and R. H. Yang, Chem. Commun., 2012, 48, 88 RSC.
- C. M. Yu, X. Z. Li, F. Zeng, F. Y. Zheng and S. Z. Wu, Chem. Commun., 2013, 49, 403 RSC.
- L. Zhou, Y. H. Lin, Z. Z. Huang, J. S. Ren and X. G. Qu, Chem. Commun., 2012, 48, 1147 RSC.
- Y. P. Shi, Y. Pan, H. Zhang, Z. M. Zhang, M. J. Li, C. Q. Yi and M. S. Yang, Biosens. Bioelectron., 2014, 56, 39 CrossRef CAS PubMed.
- H. Peng and J. T. Sejdic, Chem. Mater., 2009, 21, 5563 CrossRef CAS.
- S. J. Zhu, Q. N. Meng, L. Wang, J. H. Zhang, Y. B. Song, H. Jin, K. Zhang, H. C. Sun, H. Y. Wang and B. Yang, Angew. Chem., Int. Ed., 2013, 52, 3953 CrossRef CAS PubMed.
- Z. S. Qian, J. J. Ma, X. Y. Shan, H. Feng, L. X. Shao and J. R. Chen, Chem.–Eur. J., 2014, 20, 2254 CrossRef CAS PubMed.
- Y. Q. Zhang, D. K. Ma, Y. Zhuang, X. Zhang, W. Chen, L. L. Hong, Q. X. Yan, K. Yu and S. M. Huang, J. Mater. Chem., 2012, 22, 16714 RSC.
- Z. L. Wu, P. Zhang, M. X. Gao, C. F. Liu, W. Wang, F. Leng and C. Z. Huang, J. Mater. Chem. B, 2013, 1, 2868 RSC.
- B. Y. Han, J. P. Yuan and E. K. Wang, Anal. Chem., 2009, 81, 5569 CrossRef CAS PubMed.
- F. Pu, Z. Z. Huang, J. S. Ren and X. G. Qu, Anal. Chem., 2010, 82, 8211 CrossRef CAS PubMed.
- J. Zheng, J. S. Li, X. X. Gao, J. Y. Jin, K. M. Wang, W. H. Tan and R. H. Yang, Anal. Chem., 2010, 82, 3914 CrossRef CAS PubMed.
- C. Kar, M. D. Adhikari, A. Ramesh and G. Das, Inorg. Chem., 2013, 52, 743 CrossRef CAS PubMed.
- M. H. Lan, J. S. Wu, W. M. Liu, H. Y. Zhang, W. J. Zhang, X. Q. Zhuang and P. F. Wang, Sens. Actuators, B, 2011, 156, 332 CrossRef CAS PubMed.
- B. Liu, J. F. Wang, G. Zhang, R. K. Bai and Y. Pang, ACS Appl. Mater. Interfaces, 2014, 6, 4402 CAS.
- H. M. Lv, X. F. Yang, Y. G. Zhong, Y. Guo, Z. Li and H. Li, Anal. Chem., 2014, 86, 1800 CrossRef CAS PubMed.
- S. H. Hu, Y. W. Chen, W. T. Hung, I. W. Chen and S. Y. Chen, Adv. Mater., 2012, 24, 1748 CrossRef CAS PubMed.
- S. H. Hu, R. H. Fang, Y. W. Chen, B. J. Liao and S. Y. Chen, Adv. Funct. Mater., 2014, 24, 4144 CrossRef CAS.
Footnote |
† Electronic supplementary information (ESI) available: Details of spectral data. See DOI: 10.1039/c4tb01354a |
|
This journal is © The Royal Society of Chemistry 2015 |
Click here to see how this site uses Cookies. View our privacy policy here.