DOI:
10.1039/C5AY02494C
(Communication)
Anal. Methods, 2016,
8, 40-44
Synthesis of dopamine-mediated Cu nanoclusters for sensing and fluorescent coding†
Received
18th September 2015
, Accepted 19th November 2015
First published on 25th November 2015
Abstract
Herein, the synthesis of Cu nanoclusters (CuNCs) templated with dopamine has been first successfully established by reducing CuSO4 in an aqueous medium, and this type of nanocluster emitted cyan high-fluorescence. Subsequently, the CuNCs were employed for assaying tetracycline (TC) on the basis of the quenching of the fluorescence intensity of CuNCs once TC was introduced. The proposed analytical strategy permitted the detection of TC in a linear range of 3.6 × 10−6 to 1.0 × 10−3 mol L−1 together with a detection limit of 9.2 × 10 −7 mol L−1 at a signal-to-noise ratio of 3. Meaningfully, the CuNCs described here were further applied for fluorescent painting and coding.
Introduction
Noble metal nanoclusters (NCs) with properties regulated by their subnanometer dimensions basically consist of several to tens of atoms and exist in sizes near to the Fermi wavelength. Interestingly, the spatial confinement of free electrons in nanoclusters has provided size-tunable electronic transitions, thus facilitating their attractive fluorescence properties.1–3 Recently, nanoclusters (NCs) have been emerging as one type of promising fluorescent material owing to their obvious advantages including satisfactory water solubility, high quantum-yield, biocompatibility, excellent stability, outstanding catalytic properties, etc.4–8 which provided them with possibilities for potential applications in single-molecule imaging, biolabeling, sensing, etc.8–11 Nevertheless, the previous studies focused on Au and Ag nanoclusters; however, CuNCs have attracted considerable interest due to their unique fluorescence properties.12–15
Currently, there exist several methods for synthesizing CuNCs1,12,16 by using some surface protecting ligands such as DNA,17 proteins,18 polymers,19–21 and thiols.14 Besides, surfactants have been employed to encapsulate copper atoms thus forming CuNCs based on an electrochemical mechanism.22 Most recently, benzotriazole was applied as a template to reach the desired smaller size.23 However, these methods exhibited some drawbacks such as tedious steps, toxic reagents, complicated instrumentation and high cost. Thus, exploring simple and facile strategies for synthesizing water soluble, stable and highly quantum efficient fluorescent NCs is still desired, but even more challenging.
In this contribution, we have first successfully synthesized CuNCs stabilized with dopamine by using a one-pot approach, where CuSO4 was reduced by sodium borohydride and hydrazine hydrate (Fig. 1). Significantly, it was proved that the fluorescence of CuNCs was quenched by TC. Thereby, an analytical strategy for the detection of tetracycline has been established based on this phenomenon. Meanwhile, the CuNCs here were further employed for preparing fluorescent paints and coding.
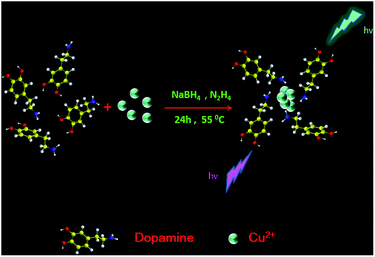 |
| Fig. 1 Schematic illustration of the synthesis of water-soluble CuNCs. | |
Experimental
Materials and measurements
All the ions (Hg2+, K+, Ag+, Zn2+, Pb2+, Eu3+, Al3+, Ca2+, Ba2+, Br−1, Mg2+, Fe3+, Cd2+, Ni2+ and I−1), copper sulfate (CuSO4), and dopamine (DOPA) as well as amino acids were obtained from Shanghai Sangon Biotechnology Co., Ltd (Shanghai, China). Sodium borohydride, tetracycline, penicillin, ampicillin, streptomycin, lincomycin, chloramphenicol and cefalexin were obtained from Sigma-Aldrich (Milwaukee, USA). Disodium hydrogen phosphate (Na2HPO4) and sodium dihydrogen phosphate (NaH2PO4), and sodium hydroxide (NaOH) were purchased from Dingguo Changsheng Biotechnology Co., Ltd (Beijing, China). Ultrapure water, 18.25 MV cm, produced with an Aquapro AWL-0502 P ultrapure water system (Chongqing, China) was employed for all experiments. All fluorescence measurements were performed on a Hitachi F-7000 fluorescence spectrophotometer (Tokyo, Japan) with the excitation slit set at a 5 nm band pass and emission at a 5 nm band pass in a 1 cm × 1 cm quartz cell. High-resolution transmission electron microscopy (HR-TEM) images were obtained by using a TECNAI G2 F20 microscope (FEI, USA) at 200 kV. Elemental and functional group analysis were performed by using a ESCALAB 250 X-ray photoelectron spectrometer and a Fourier transform infrared spectrometer (Tokyo, Japan), respectively. A Fangzhong pHS-3C digital pH meter (Chengdu, China) was applied to measure the pH values of the aqueous solutions and a vortex mixer QL-901 (Haimen, China) was employed for blending the solution. The thermostatic water bath (DF-101s) was purchased from Gongyi Instrument Co., Ltd (Gongyi, China).
Synthesis of CuNCs
Basically, as a typical experiment, 5 mL of dopamine solution (10 mM) was added into 5 mL of CuSO4 solution (10 mM). After 2 min stirring, 200 μL of NaBH4 solution (0.25 mol L−1) was added, and the mixture was incubated under vigorous stirring at 25 °C in a water bath for 5 min. Next, N2H4·H2O (200 μL) was introduced to the mixture under vigorous stirring and further incubated at 55 °C in a water bath for 12 h. Again, the solution was incubated overnight at room temperature. After the centrifugation (10
000 rpm, 10 min), the supernatant containing fluorescent CuNCs was collected. Then, this aqueous solution was filtered using a 0.22 mm filter membrane to remove the larger products. Finally, the fluorescent carbon dots were collected by dialysis against deionized water though a dialysis membrane (300 MWCO) for 24 h. The CuNCs obtained here were stored in the dark at 4 °C for further applications.
Detection of tetracycline
Firstly, 50 μL of CuNCs and 50 μL of PBS buffer (0.2 M, pH 7.4) were pipetted into a 1.5 mL vial. Subsequently, an appropriate volume of tetracycline solution was introduced followed by dilution to 500 μL with Milli-Q purified water and thorough vortex-mixing. The mixture was then incubated to react at 60 °C for 10 min and transferred for fluorescence measurements. To evaluate the interference in pharmaceutical preparations, a variety of metal ions (Hg2+, K+, Ag+, Zn2+, Pb2+, Eu3+, Al3+, Ca2+, Ba2+, Br−, Mg2+, Fe3+, Cd2+, Ni2+, and I−) and amino acids (Val, Lys, Phe, Tyr, Ser, Pro, Glu, and Ala) were respectively introduced with the identical concentration of 400 μM. Similarly, effects originating from 10 mM of each antibiotic (penicillin, ampicillin, streptomycin, lincomycin, cephalexin and chloramphenicol) were further explored towards the selectivity of the current method.
Analysis of milk samples
Briefly, proteins in the milk samples were first removed by adding 1% trichloroacetic acid followed by sonication for 20 min. After filtering through a 0.22 μm membrane to remove lipids, the supernatant was collected into 3 tubes and spiked with TC solutions (10 μM, 13 μM 15 μM, 18 μM and 20 μM).
Results and discussion
Characterization of CuNCs
To elucidate the fluorescence mechanism of the current CuNCs, the maximum excitation and emission spectra were recorded at 425 nm and 480 nm, respectively. As shown in Fig. 2A, the CuNC solution emitted obvious cyan fluorescence (photograph II) under UV light (365 nm) while appearing yellowish and transparent under daylight (photograph I). Meanwhile, the as-synthesized CuNCs excited by using a 850 nm laser showed the maximum up-converted emission peak at 425 nm (Fig. 2B), suggesting their infrared up-conversion properties. The data in Fig. 2C indicate that the fluorescence originated from the CuNCs rather than CuSO4 or dopamine. To explore the surface groups of this CuNCs, the spectra of Fourier transform infrared spectroscopy (FTIR) were recorded. As Fig. 2D reveals, there were O–H, N–H, C–N, and C
O groups on the surface of the CuNCs.
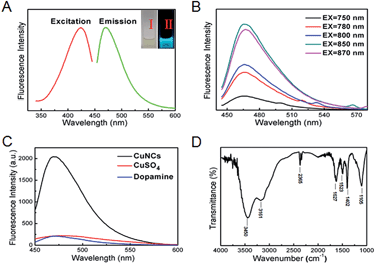 |
| Fig. 2 (A) Fluorescence excitation and emission spectra of CuNCs; (B) up-conversion fluorescence spectra of CuNCs upon varying excitation wavelengths; (C) fluorescence spectra of CuNCs, dopamine and CuSO4, respectively; (D) FTIR spectrum of the as-prepared CuNCs. | |
Toward the purpose of gaining insight into components of the current CuNCs, XPS survey spectra were provided. As shown in Fig. 3A, six major peaks of S(1s), C(1s), N(1s), Na, O(1s), Cu(2p), and Na(1s) obviously emerged in the spectrum, indicating that CuNCs prepared here were mainly composed of C, O, N, Cu, and Na. For this, XPS analysis was performed for confirming the oxidation state of Cu in the CuNC samples (Fig. 3B). Two prominent peaks observed at 953.5 and 934.2 eV were assigned to Cu 2p1/2 and Cu 2p3/2, which were characteristic peaks due to Cu0. The absence of the peak referring to Cu2+ at 942 eV indicated that the CuNCs did not contain Cu2+. As shown in HR-TEM images (Fig. 3C and D), CuNCs obtained here existed as a majority population at the size of 3 nm and no aggregation emerged. As revealed (Fig. S1A–C, ESI†), the fluorescence intensities of CuNCs scarcely exhibited alteration along with time-lapse and varying organic solvents, demonstrating the satisfactory stability of CuNCs. To address whether the CuNCs showed an excitation-dependent emission character or not, further exploration was provided. And the emission peak at 480 nm did not shift, describing their excitation-independent emission character (Fig. S1D, ESI†).
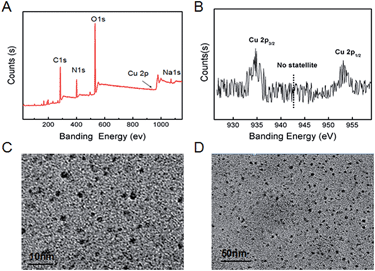 |
| Fig. 3 (A) and (B) HR-TEM images of CuNCs with different scales; (C) XPS of CuNCs thus obtained; (D) amplified XPS of Cu 2p electrons. | |
Detecting TC and optimizing the conditions
Next, the fluorescence intensity of the CuNCs at 480 nm decreased obviously once 1 mM TC was introduced (Fig. 4A). To detect TC effectively, conditions including pH, reaction time, temperature and buffers were optimized. The fluorescence intensity of CuNCs was gradually increased as the pH values varied from 2 to 11 (Fig. S2A, ESI†), indicating that altering pH showed an effect on detection of TC. Thus, a pH of 7.4 was chosen as one of the optimal conditions. As shown in Fig. S2B (ESI†), the (F0 − F/F0) values in different buffers were similar. In view of the reasonable and stable characteristics of phosphate buffer saline (PBS), we employed PBS buffer for assaying TC. After investigating the effect of temperature and the corresponding time, we chose 60 °C (Fig. S2C, ESI†) and 10 min (Fig. S2D, ESI†) to serve as the optimal conditions during the following experiments.
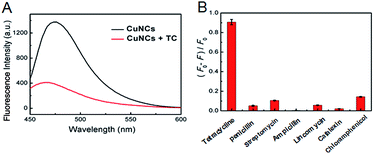 |
| Fig. 4 (A) Fluorescence spectra of CuNCs in the absence (black) and presence (red) of 1 mM tetracycline; (B) fluorescence decrease of CuNCs in the presence of tetracycline, penicillin, ampicillin, streptomycin, lincomycin, and cefalexin, respectively. | |
Selectivity
Again, a dramatic decrease of the fluorescence has been observed for CuNCs upon the addition of TC as shown in Fig. 4B. In striking contrast, no obvious decrease was found by the addition of other antibiotics into the CuNCs, revealing the satisfactory selectivity of CuNCs for assaying TC. Detection of TC was based on the fluorescence decrease of CuNCs, likely due to the interactions between the surface groups of CuNCs and TC, thereby facilitating energy trapping of CuNCs by forming new bonds.
Detection of TC by using CuNCs
The fluorescence intensity of the CuNCs at 480 nm decreased obviously once TC was introduced. In the mechanism, the zeta potentials of CuNCs with negative charge were −36.31 ± 2.14 mV. After interacting with TC, the zeta potentials changed to −31.23 ± 1.98 mV (Table S1†), indicating that the TC interacted with the surface groups of the CuNCs and further facilitated the energy trapping of CuNCs by forming new bonds, which lead to the obvious fluorescence decrease.24,25 As shown in Fig. 5A and B, there was no obvious variation of fluorescence signals while introducing common metal ions or amino acids into the mixture of CuNCs with TC, describing that the coexisting foreign substance barely showed an effect on assaying TC. Next, the fluorescence intensity decrease (F0/F) versus the logarithmic plots of TC concentrations displayed a linear range of 3.6 μM–1 mM (Fig. 5C and D). Meanwhile, the corresponding detection limits were 0.92 μM at a signal-to-noise ratio of 3. Compared with other sensors for assaying TC,25–27 the results demonstrated an efficient and facile method.
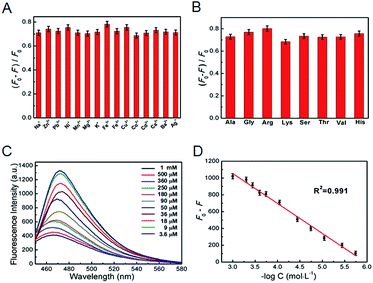 |
| Fig. 5 Influence of coexisting metal ions (A) and amino acid (B) on the fluorescence intensity (F0 − F/F0) of CuNCs in the presence of TC; (C) fluorescence emission spectra of CuNCs in the presence of various concentrations of TC; (D) plot of the fluorescence intensity decrease (F0 − F) versus the logarithm of concentrations of TC added. | |
Detection of TC in milk samples
The as-synthesized CuNCs are stable in real samples (Fig. S3, ESI†). To explore the practicality of the proposed approach, standard recovery experiments of milk samples were performed (Table 1). As listed, the recoveries of all samples were 107%, 94.6%, 95.3%, 93.3% and 98.5%.
Table 1 The recovery of TC in supplemented milk samples
Sample |
TC spiked (10−5 M) |
TC measured (10−5 M) |
Recovery (%) |
1 |
1.0 |
1.07 |
107.00 |
2 |
1.3 |
1.23 |
94.62 |
3 |
1.5 |
1.43 |
95.33 |
4 |
1.8 |
1.68 |
93.33 |
5 |
2.0 |
1.97 |
98.50 |
Fantastic applications
Benefiting from their excellent fluorescence properties, CuNCs were further employed for interesting applications. As shown in Fig. 6, the photographs suggested that fluorescent CuNCs described here could play a role in text encryption and fluorescent painting.
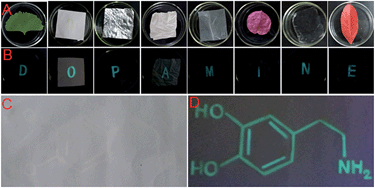 |
| Fig. 6 Photos of letters directly written on various materials (from left to right: green leaf, paper, tinfoil, rubber, plastic, flower, plastic wrap, and red leaf) by using CuNCs as ink with a brush pen under daylight (A) and UV light (B); fluorescent coding of the structural formula by using CuNCs under daylight (C) and UV light (D). | |
Conclusions
In summary, we have synthesized CuNCs stabilized with dopamine via a facile method. To be specific, the CuNCs existed with an average size of 3 nm, and emitted cyan fluorescence. Significantly, we have employed the current CuNCs for simple, selective, sensitive and economical quantifications of TC. Detection of TC was based on the fluorescence decrease of CuNCs, likely due to the interactions between the surface groups of CuNCs and TC, thereby facilitating the energy trapping of CuNCs by forming new bonds. In addition, this type of CuNC described here was successfully applied for fluorescent painting and coding.
Acknowledgements
We gratefully acknowledge the financial support from the Science Foundation of Southwest University (SWU114053), Natural Science Foundation Project of CQ CSTC (cstc2013jcyjA10117), Innovative Research Project for Postgraduate Students of Chongqing (CYS14049), and Fundamental Research Funds for the Central Universities (XDJK2015A005, XDJK2016D033).
References
- J. Xie, Y. Zheng and Y. Ying, J. Am. Chem. Soc., 2009, 131, 888–889 CrossRef CAS PubMed.
- I. Diez and R. H. A. Ras, Nanoscale, 2011, 3, 1963–1970 RSC.
- Z. Luo, X. Yuan, Y. Yu, Q. Zhang, D. T. Leong, J. Y. Lee and J. Xie, J. Am. Chem. Soc., 2012, 134, 16662–16670 CrossRef CAS PubMed.
- H. Liu, Y. Wang, L. H. Zhang, Y. Shao and B. Zheng, Mater. Lett., 2015, 139, 265–267 CrossRef CAS.
- S. A. Patel, C. I. Richards, J. C. Hsiang and R. M. Dickson, J. Am. Chem. Soc., 2008, 130, 11602–11605 CrossRef CAS PubMed.
- H. Zhang, X. Huang, L. Li, G. W. Zhang, I. Hussain, Z. Li and B. Tan, Chem. Commun., 2012, 48, 567–569 RSC.
- F. Yao, X. Yuan, Y. Yu, Y. Yu, J. Xie and J. Y. Lee, J. Am. Chem. Soc., 2015, 137, 2128–2136 CrossRef PubMed.
- Q. Yao, Y. Yu, X. Yuan, Y. Yu, D. Zhao, J. Xie and J. Y. Lee, Angew. Chem., 2015, 54, 184–189 CrossRef CAS PubMed.
- L. Balogh and D. A. Tomalia, J. Am. Chem. Soc., 1998, 120, 7355–7356 CrossRef CAS.
- H. Cao, Z. Chen, H. Zheng and Y. Huang, Biosens. Bioelectron., 2014, 62, 189–195 CrossRef CAS PubMed.
- Y. Yu, Z. Luo, D. M. Chevrier, D. T. Leong, P. Zhang, D. E. Jiang and J. Xie, J. Am. Chem. Soc., 2014, 136, 1246–1249 CrossRef CAS PubMed.
- D. J. Bradwell, S. Osswald, W. F. Wei, S. A. Barriga, G. Ceder and D. R. Sadoway, J. Am. Chem. Soc., 2011, 133, 19971–19975 CrossRef CAS PubMed.
- M. Fernandez-Ujados, L. Trapiella-Alfonso, J. M. CostaFernandez, R. Pereiro and A. Sanz-Medel, Nanotechnology, 2013, 24, 495601 CrossRef PubMed.
- X. Yuan, Z. Luo, Q. Zhang, X. Zhang, Y. Zheng, J. Y. Lee and J. Xie, ACS Nano, 2011, 5, 8800–8808 CrossRef CAS PubMed.
- X. Yang, Y. Feng, S. Zhu, Y. Luo, Y. Zhuo and Y. Dou, Anal. Chim. Acta, 2014, 847, 49–54 CrossRef CAS PubMed.
- X. Jia, X. Yang, J. Li, D. Y. Liab and E. Wang, Chem. Commun., 2014, 50, 237–239 RSC.
- A. Rotaru, S. Dutta, E. Jentzsch, K. Gothelf and A. Mokhir, Angew. Chem., Int. Ed., 2010, 49, 5665–5667 CrossRef CAS PubMed.
- N. Goswami, A. Giri, M. S. Bootharaju, P. L. Xavier, T. Pradeep and S. K. Pal, Anal. Chem., 2011, 83, 9676–9680 CrossRef CAS PubMed.
- A. Ganguly, I. Chakraborty, T. Udayabhaskararao and T. Pradeep, J. Nanopart. Res., 2013, 15, 1–7 CrossRef.
- H. Kawasaki, Y. Kosaka, Y. Myoujin, T. Narushima, T. Yonezawa and R. Arakawa, Chem. Commun., 2011, 47, 7740–7742 RSC.
- M. Zhao, L. Sun and R. M. Crooks, J. Am. Chem. Soc., 1998, 120, 4877–4878 CrossRef CAS.
- N. Vilar-Vidal, M. C. Blanco, M. A. Lopez-Quintela, J. Rivas and C. Serra, J. Phys. Chem. C, 2010, 114, 15924–15930 CAS.
- K. Salorinne, X. Chen, R. W. Troff, M. Nissinen and H. Hakkinen, Nanoscale, 2012, 4, 4095–4098 RSC.
- J. Hou, J. Yan, Q. Zhao, Y. Li, H. Ding and L. Ding, Nanoscale, 2013, 5, 9558–9561 RSC.
- J. Lin and Q. Wang, RSC Adv., 2015, 5, 27458–27463 RSC.
- D. Chen, D. Yao, C. Xie and D. Liu, Food Control, 2014, 42, 109–115 CrossRef CAS.
- H. Tan and Y. Chen, Sens. Actuators, B, 2012, 173, 262–267 CrossRef CAS.
Footnote |
† Electronic supplementary information (ESI) available. See DOI: 10.1039/c5ay02494c |
|
This journal is © The Royal Society of Chemistry 2016 |
Click here to see how this site uses Cookies. View our privacy policy here.