Field evaluation of the effectiveness of three industrial by-products as organic amendments for phytostabilization of a Pb/Zn mine tailings†
Received
20th September 2015
, Accepted 4th November 2015
First published on 4th November 2015
Abstract
Although the potential of industrial by-products as organic amendments for phytostabilization has long been recognized, most of the previous studies addressing this issue have been laboratory-based. In this study, a field trial was conducted to evaluate the effectiveness of three industrial by-products [sweet sorghum vinasse (SSV), medicinal herb residues (MHR) and spent mushroom compost (SMC)] as organic amendments for phytostabilization of abandoned Pb/Zn mine tailings. Our results showed the following: (i) when compared to the control tailings, the mean concentrations of diethylene-triamine-pentaacetic acid (DTPA)-extractable Cd, Cu, Pb and Zn in SSV, MHR and SMC treatments decreased by 20.8–28.0%, 41.6–49.1%, 17.7–22.7% and 9.5–14.7%, respectively; (ii) the mean values of organic C, ammonium-N and available P in SSV, MHR and SMC treatments increased by 1.7–2.8, 10.8–14.9 and 3.9–5.1 times as compared with the mine tailings; and (iii) the addition of SSV, MHR and SMC significantly enhanced soil respiration and microbial biomass being 1.5–1.8 and 1.3–1.6 fold higher than those in the control tailings. There were no significant differences in soil biochemical properties among the plots amended with these by-products, suggesting that they were almost equally effective in improving the biochemical conditions of the tailings. In addition, the application of these amendments promoted seed germination, seedling growth, and consequently increased the vegetation cover and its biomass. Moreover, concentrations of Cd, Cu, Pb and Zn in above-ground parts of the plants were below the toxicity limit levels for animals. The results obtained in this field study confirmed that the three organic-rich industrial by-products could be used as amendments for phytostabilization of some types of mine tailings.
Environmental impact
Laboratory-based research has shown that the industrial by-products (sweet sorghum vinasse, medicinal herb residues and spent mushroom compost) were effective in reducing the metal bioavailability and restoring the ecological functions of Pb/Zn mine tailings. However, field evaluation of the effectiveness of these by-products is necessary before they can be applied on a full scale. In the present field study, we found that the three organic-rich industrial by-products were approximately equally effective in reducing the levels of bioavailable metals in the mine tailings, increasing the soil nutrient status, and enhancing soil respiration, microbial biomass and enzyme activities. In addition, the application of these amendments increased the vegetation cover and biomass, and reduced the metal concentrations in plant tissues. Our results provided strong evidence that the three industrial by-products could be used as organic amendments for phytostabilization of mine tailings.
|
1 Introduction
Mine tailings are of environmental concern in post-mining landscapes since they present a permanent threat to surrounding populations and ecosystems. The remediation of mine tailings remains a challenge globally. Traditional remediation practices used in mining areas, such as excavation, transport and landfilling are not feasible and appropriate for mine tailings due to their frequent high concentrations of heavy metals and the scale of storage facilities (area, depth, and volume).1 The in situ stabilization technique is currently being explored with the aim of minimizing adverse environmental impacts of the tailings by increasing the stability of land surfaces, impairing mobility and toxicity of metals and reducing wind and water erosion.2
Stabilization techniques are generally based on physical, chemical or phytostabilization.3 Physical stabilization refers to the use of innocuous materials to cover the unstable mine tailings. Chemical stabilization aims to form a crust or surface layer over the tailings by adding chemical agents. Phytostabilization establishes a permanent vegetation cover on the mine tailings to reduce surface erosion and to prevent contaminant dispersion. In many cases, the widespread application of physical or chemical stabilization techniques is limited by the availability of suitable materials and high costs.2,4 In contrast, phytostabilization has received a growing amount of interest and is emerging to be a promising solution for mine tailings due to its several advantages such as stabilization, pollution control and visual improvement.5,6
Although phytostabilization is desirable, direct establishment of vegetation on the barren mine tailings is limited due to unfavorable conditions – particularly high concentrations of metals, lack of normal soil structure, low/no organic matter or macronutrients.6 To overcome these limitations, amendments may be added. Among the suitable amendments for phytostabilization, organic-rich materials appear to be excellent candidates. The use of organic amendments in mine tailings reclamation offers the following advantages: (1) improvement of the physical and chemical nature of the tailings, especially improving the water- and nutrient-holding capacities; (2) supply of plant nutrients in a slow-release form, facilitating early plant establishment; (3) in situ immobilization of heavy metals by reducing their bioavailability and phytotoxicity; and (4) re-establishment of microbial populations, eventually restoring the ecological function to the tailings.4
Various organic materials have been proposed and tested for phytostabilization of heavy metals in contaminated soils, including agricultural and industrial by-products. Alvarenga et al.7,8 employed three industrial by-products (sewage sludge, municipal solid waste compost and garden waste compost) as immobilizing agents for phytostabilization of a highly acidic metal-contaminated soil, showed that all three residues significantly corrected soil acidity, decreased Cu, Pb and Zn mobile fractions, enhanced soil enzyme activities and increased plant biomass. Chiu et al.9 reported that the application of industrial by-products (manure compost and sewage sludge) to Pb/Zn and Cu mine tailings increased N, P and K contents of the tailings, decreased DTPA-extractable Pb, Zn concentrations in Pb/Zn tailings and DTPA-extractable Cu concentrations in Cu tailings, and ultimately led to a reduction in heavy metal uptake and accumulation by the grasses Vetiveria zizanioides and Phragmites australis. Lee et al.2 found that the addition of iron-rich industrial by-products (limestone, red mud and furnace slag) to arsenic and metal-contaminated agricultural soils not only lowered the availability of trace elements, but also improved the soil microbial community structure and function. Our earlier attempt to remediate Pb/Zn tailings by adding industrial by-products (sweet sorghum vinasse, medicinal herb residues and spent mushroom compost) has been proved to be efficient in decreasing extractable metal concentrations, enhancing enzyme activities and reducing metal concentrations in plant tissues.10 However, in most cases, the effectiveness of industrial by-products proposed in the literature was tested under laboratory conditions. Many studies have noted that the greenhouse scenario cannot represent the real field condition and it is difficult to apply the results into practice.11,12 Therefore, field evaluations are essential before the application of these materials at the field-scale or in real situations.
The present work focused on the restoration of a vegetation cover for Pb/Zn mine tailings through phytostabilization combining organic industrial by-products and metal-tolerant plants. A field study was carried out at an abandoned Pb/Zn mine tailings pond to evaluate the effectiveness of three freely-available industrial by-products (SSV: sweet sorghum vinasse; MHR: medicinal herb residues and SMC: spent mushroom compost) for phytostabilization of Pb/Zn mine tailings. We hypothesized that the three amendments might improve the physico-chemical properties of the tailings, reduce heavy metal availability and enhance microbial activity, thereby enhancing plant establishment and growth. To test our hypothesis, we studied soil chemical properties (available heavy metals, macronutrients), microbial activities, plant growth and metal accumulation in plant tissues. Relationships between soil properties and plant parameters were further analyzed to demonstrate the remediation efficacy of these industrial by-products in phytostabilization.
2 Materials and methods
2.1 Study site
The Huayuan Pb/Zn mine (28°06′N, 109°11′E) is located in Xiangxi Tujia and Miao Autonomous District, Hunan Province, China. This mining area has a middle subtropical mountainous climate with a mean annual temperature of 16.7 °C and an annual average precipitation of 1421 mm. The region has an abundant reserve of manganese and zinc. Mining and processing activities have generated large quantities of tailings deposited on the ground with over 100 tailings sites abandoned (data from the local Environmental Protection Bureau). The tailings pond studied had been abandoned for about two years. The tailings surface was dry and completely devoid of vegetation. The main physico-chemical properties of the tailings were detailed in our previous study.10
2.2 Experimental design
The experiment was performed in a plot (30 m × 20 m) in the center of the abandoned Pb/Zn tailings pond. The experimental plot was divided into 20 subplots of 4 m2 (2 m × 2 m), leaving a corridor of 2 m between subplots as a barrier to avoid interactions between them. Three industrial by-products (SSV, MHR and SMC) were used as organic amendments and detailed information about them was given in our previously reported pot experiment.10 The three specific industrial by-products were chosen since they are widely available at a local level, have a low cost and are easy to apply. These were applied at 12 kg per subplot (equivalent to 30 t ha−1, a rate based on the results of our pot experiment)10 and incorporated manually with a hoe into the upper layer of tailings (0–30 cm depth). The subplots were arranged according to a complete randomized block design with four replicates per treatment. Two control treatments were also established: tailings without amendment (tailings) and tailings with a local ‘normal’ topsoil (30 t ha−1, NTS). Topsoil was selected for comparison because it has been used as an amendment by local residents to cover mine tailings. Sowing took place after the amendment addition and equilibration for two months. A grass seed mix of Lolium perenne, Cynodon dactylon, Medicago sativa and Dendranthema indicum (5/g m2 for L. perenne, C. dactylon and M. sativa; 2.5/g m2 for D. indicum, according to the seed germination in the pot experiment) was sown by hand. Plants were grown under natural conditions and neither agricultural practices nor irrigation was employed.
2.3 Soil sampling and analysis
Soil samples were collected from all experimental subplots at the time of sowing (0) and then at 3, 6, 9 and 12 months after planting. In each case, 5 regularly distributed soil cores (5 cm diameter, 25 cm depth, using a manual stainless steel soil auger) were taken from each subplot to prepare a composite sample. The soil samples were immediately transported to the laboratory and divided into two subsamples. One subsample was air-dried, passed through a 2 mm sieve and subjected to chemical analysis; the other fresh subsample was sieved to 2 mm and then stored at 4 °C for microbial analysis.
2.3.1 Chemical analysis.
Organic C (OC) was analyzed by dichromate oxidation and titration with ferrous sulphate.13 Ammonium-N (NH4+-N) was extracted with potassium chloride and measured by colorimetric N determination.14 Available P (AP) was extracted with sodium bicarbonate and estimated according to the molybdenum blue method.15 Soil bioavailable metals were extracted using a mixture of 5 mM DTPA (diethylene-triamine-pentaacetic acid), 10 mM CaCl2 and 100 mM triethanolamine at pH 7.3 (ref. 16) and determined by Inductively-Coupled Optical Emission Spectrometry (ICP-OES, iCAP6300, Thermo Electron, USA). Quality assurance of metal analysis was performed using added certified reference materials (provided by Beijing Shijiaoke Biotechnology, China) in the extracted solution (recovery ratio 84–116%). The detection limits of Cd, Pb and Zn were 0.001, 0.001 and 0.003 mg L−1, respectively.
2.3.2 Microbial analysis.
Basal soil respiration was measured according to the method of Anderson:17 the field-moist soil was incubated in an air-tight sealed jar at 25 °C for 24 h in the dark. CO2 produced during the test was absorbed in 0.05 M NaOH and quantified by titration with 0.1 M HCl. Soil microbial biomass C was determined based on the modification of the chloroform fumigation extraction method of Vance et al.18 Ten gram samples of field moist soil were fumigated with chloroform for 7 days and extracted for 1 h in 50 mL K2SO4. Organic C in the extracts was oxidized by dichromate and titration with FeSO4. Microbial C was calculated as the difference between fumigated and non-fumigated samples and corrected with a KEC value of 0.38. Dehydrogenase activity was measured using the method of Thalmann.19 β-Glucosidase activity was determined as proposed by Hoffmann and Dedeken.20 Urease activity was measured using a buffered method described by Kandeler and Gerber.21 Phosphatase activity was determined following the method of Tabatabai and Bremner.22 The detailed experimental procedures for soil enzyme analysis were described previously in our earlier study.10
2.4 Plant sampling and analysis
Plant investigations and samplings were taken on two occasions, at 6 and 12 months after sowing. Vegetation cover was estimated by the percentage of the subplots covered by grasses. Plant sampling involved clipping grass at 5 mm above ground within a quadrat (0.5 m × 2 m) in each subplot. The root material was not removed in order not to disrupt the established vegetation. All plant species were listed, washed thoroughly with tap water, rinsed three times with deionized water and, finally oven-dried at 80 °C for 48 h for determining biomass production and metal concentrations. Approximately 0.5 g of finely-ground plant samples were digested with a mixture of concentrated HNO3 and concentrated HClO4 at 5
:
1 (v/v).23 The concentrations of Cd, Cu, Pb and Zn in the plant materials were determined by ICP-OES analysis of the digests. A certified reference plant material (GBW07405) obtained from the National Center for Standard Materials was used to verify the accuracy of metal determination. The recovery ratios were: 93 ± 6% for Cd, 89 ± 7% for Cu, 88 ± 5% for Pb and 97 ± 3% for Zn, respectively.
2.5 Statistical analysis
All statistical analyses were conducted using the SPSS 16.0 for Windows. Datasets were checked for homogeneity of variance and normality (Kolmogorov–Smirnov test) and, when necessary, log-transformed. One-way ANOVA was carried out to compare the means of different treatments, followed by multiple comparisons using the least significant difference test. The level of significance was set at p < 0.05. Pearson's correlation coefficients were calculated between plant parameters and soil biochemical properties. Two levels of significance were considered, p < 0.05 and p < 0.01. A multivariate approach was applied to explore the relationship between soil biochemical properties and plant parameters using canonical correspondence analysis (Canoco 4.5 for Windows).24 A summary diagram was prepared in which soil biochemical variables were represented as arrows: the length of these arrows indicated the relative importance of the soil factor in explaining the variation in plant development and the angle between the arrows indicated the degree to which they were correlated.
3 Results
3.1 Effects of industrial by-products on heavy metal availability
The DTPA-extractable metal concentrations with different industrial by-products are presented in Fig. 1. In the tailings, the DTPA-extractable metal concentrations were within the range of 0.97–0.99 mg kg−1 for Cd, 6.11–6.78 mg kg−1 for Cu, 46.36–47.89 mg kg−1 for Pb and 96.12–100.17 mg kg−1 for Zn, respectively. The addition of SSV, MHR and SMC significantly decreased DTPA-extractable metal concentrations. The mean values of DTPA-extractable Cd, Cu, Pb and Zn in SSV, MHR and SMC treatments decreased by 20.8–28.0%, 41.6–49.1%, 17.7–22.7% and 9.5–14.7% as compared to the control tailings. No significant differences were found between the two controls (tailings and NTS). In addition, DTPA-extractable Cd, Cu, Pb and Zn in all the treatments remained constant over one-year of remediation.
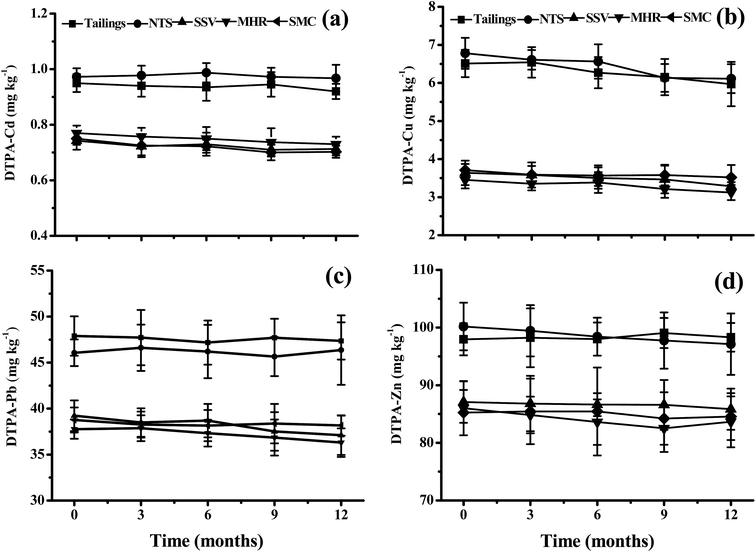 |
| Fig. 1 DTPA-extractable Cd, Cu, Pb and Zn concentrations in the tailings with different amendments and remediation time (mean ± SE, n = 4). | |
3.2 Effects of industrial by-products on nutrient accumulation
Fig. 2 shows the accumulation of major nutrients in different treatments. As expected, the addition of SSV, MHR and SMC significantly increased OC, NH4+-N and AP relative to the tailings and NTS treatments. When compared to the control tailings, the mean values of OC, NH4+-N and AP in SSV, MHR and SMC treatments increased by 1.7–2.8, 10.8–14.9 and 3.9–5.1 times, respectively. A slightly increasing trend in OC, NH4+-N and AP in SSV, MHR and SMC treatments was observed as the remediation time progressed. For example, OC contents in SSV, MHR and SMC treatments were 7.18, 7.02 and 7.29 g kg−1 at the initial sampling and increased to 9.36, 9.55 and 10.35 g kg−1 at the last sampling. In contrast, the contents of OC, NH4+-N and AP in tailings and NTS remained unchanged.
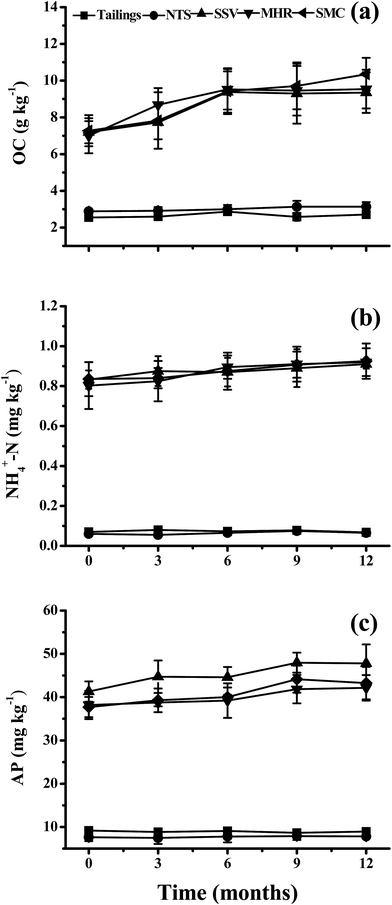 |
| Fig. 2 The accumulation of major nutrients in the tailings with different amendments and remediation time (mean ± SE, n = 4). | |
3.3 Effects of industrial by-products on microbial activity
The soil respiration, microbial biomass and enzyme activities with different industrial by-products are shown in Fig. 3 and 4. In all cases, the two controls (tailings and NTS) showed very low mean values for soil respiration and microbial biomass and the added industrial by-products (SSV, MHR and SMC) significantly enhanced soil respiration and microbial biomass, being 1.5–1.8 and 1.3–1.6 fold higher than those in the control tailings. An appreciable trend of increasing microbial biomass with time was observed, whereas the mean values of soil respiration remained constant (Fig. 3). Similarly, the addition of SSV, MHR and SMC also significantly increased soil enzyme activities in comparison to the two controls. Overall, the levels of the four soil enzyme activities were similar in the three organic treatments at each sampling time and there was an appreciably increasing trend in dehydrogenase, β-glucosidase, urease and phosphatase activities with time in SSV, MHR and SMC treatments (Fig. 4).
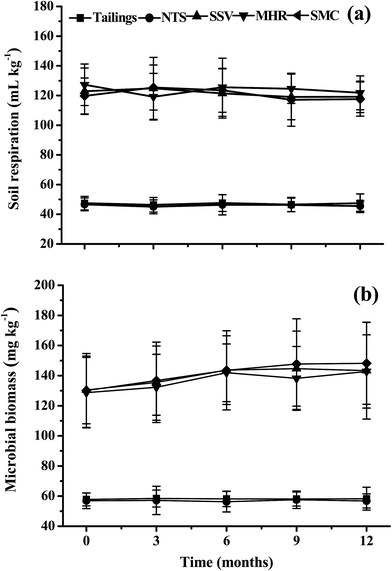 |
| Fig. 3 Soil microbial activity and biomass in the tailings with different amendments and remediation time (mean ± SE, n = 4). | |
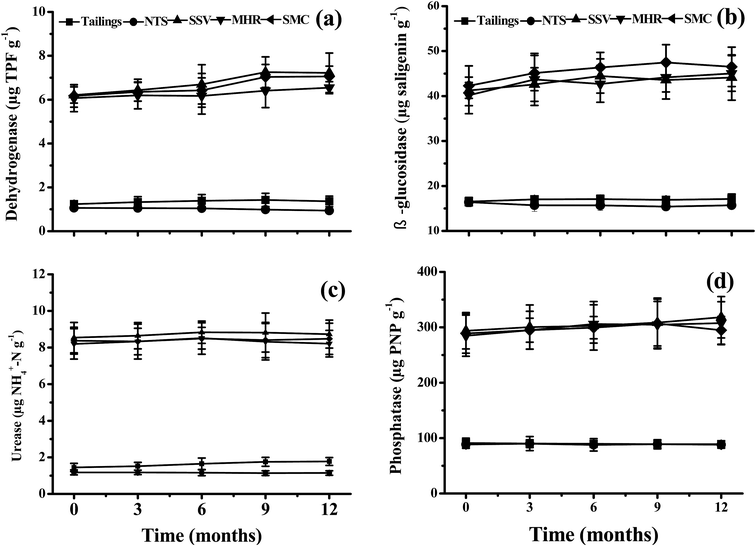 |
| Fig. 4 Soil enzyme activity in the tailings with different amendments and remediation time (mean ± SE, n = 4). | |
3.4 Effects of industrial by-products on vegetation cover, biomass and heavy metal accumulation in the plant tissues
The vegetation cover was assessed as the average of the two investigations and the biomass yield of each species was the sum of the two harvests. Fig. 5a shows that the vegetation cover was about 25% and 35% for the two control subplots, and reached 84%, 79% and 86% at SSV, MHR and SMC subplots, respectively. For L. perenne and C. dactylon, the addition of SSV, MHR and SMC led to significant increase in the shoot biomass yields with 4.2–5.6 and 15.7–17.3 fold greater than those in the tailings (Fig. 5b). No seeds of M. sativa and D. indicum could germinate in the control subplots (tailings and NTS); therefore, there were no biomass data for them. The addition of SSV, MHR and SMC triggered the seed germination and seeding growth of M. sativa and D. indicum. Due to the similarity of metal concentrations in shoots of L. perenne and C. dactylon in the two harvests of each harvest, only data from the second harvest (12 month) are presented (Table 1). The addition of SSV, MHR and SMC significantly reduced the concentrations of Cd, Cu, Pb and Zn in the shoots of L. perenne and C. dactylon in comparison with the tailings and NTS treatments. However, no significant differences were found in shoot metal concentrations between the three organic treatments. The metal concentrations (Cd, Cu, Pb and Zn) in the shoots of M. sativa and D. indicum are presented in Table S1.†
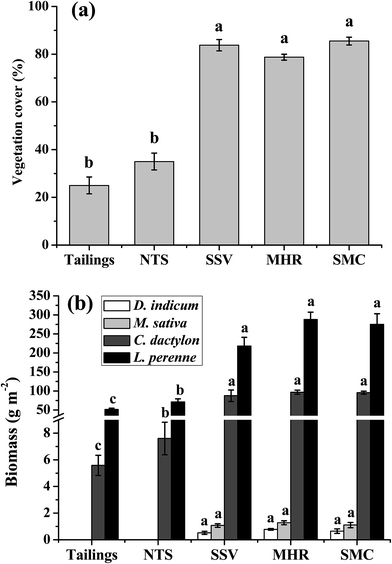 |
| Fig. 5 Vegetation cover and plant biomass in the tailings with different amendments and remediation time (mean ± SE, n = 4). Different letters in the bar indicate a significant difference at p < 0.05 according to LSD tests. | |
Table 1 Concentrations of Cd, Cu, Pb and Zn in the shoots of L. perenne and C. dactylon grown in different substrata (mean ± SE, n = 4)
Treatments |
L. perenne
|
C. dactylon
|
Cd |
Cu |
Pb |
Zn |
Cd |
Cu |
Pb |
Zn |
Different letters in the same column indicate a significant difference at p < 0.05 according to LSD tests. |
CK |
16.94 ± 3.06a |
16.44 ± 0.97a |
101.06 ± 22.29a |
357.66 ± 39.42a |
10.36 ± 2.65a |
20.44 ± 1.62a |
93.82 ± 12.92a |
418.76 ± 28.21a |
NTS |
12.03 ± 1.5b |
14.53 ± 1.61a |
88.14 ± 11.25a |
242.52 ± 45.07b |
7.09 ± 2.56a |
17.03 ± 1.4b |
89.62 ± 6.6b |
330.88 ± 34.22b |
SSV |
2.77 ± 0.84c |
8.91 ± 0.55b |
29.33 ± 5.72b |
52.79 ± 8.4c |
3.19 ± 0.51b |
10.02 ± 0.94c |
20.77 ± 4.50c |
78.58 ± 9.55c |
MHR |
2.42 ± 0.34c |
8.42 ± 1.05b |
24.37 ± 5.58b |
68.8 ± 1.09c |
4.30 ± 0.88b |
9.17 ± 0.98c |
20.06 ± 2.33c |
86.89 ± 17.13c |
SMC |
2.08 ± 0.45c |
9.58 ± 1.32b |
23.15 ± 5.29b |
62.93 ± 4.97c |
4.93 ± 0.64b |
10.83 ± 1.13c |
17.28 ± 1.17c |
87.73 ± 18.12c |
3.5 Relationship between plant parameters and soil biochemical properties
Pearson's correlation coefficients between plant parameters and soil biochemical properties are listed in Table 2. The vegetation cover and biomass were positively correlated with soil nutrient elements (OC, NH4+-N and AP) and microbial parameters (soil respiration, microbial biomass and enzyme activities). Significant negative correlations were observed between DTPA-extractable metal concentrations and vegetation cover and biomass. The metal concentrations in plants were positively correlated with soil DTPA-extractable metal concentrations and negatively correlated with soil nutrient elements and microbial parameters. In general, the significances were at lower significance levels (p < 0.01). Canonical correspondence analysis (CCA) was carried out to determine how soil biochemical properties influenced the plant development. CCA revealed that all the tailings subplots clustered into two groups attributed to the variation in soil and plant parameters (Fig. 6). Axes 1 and 2 were found to explain 76.6% and 17.6% of the overall variance and plant–soil correlations for both axes were 0.96, indicating a strong correlation between plant parameters and soil biochemical properties. The two control subplots were located on the negative side of axis 1 which indicated that they had a strong correlation with soil DTPA-extractable metal concentrations. In contrast, the subplots added SSV, MHR and SMC all positioned in the positive side of axis 1, suggesting that they were strongly correlated with soil nutrient elements and microbial parameters.
Table 2 Pearson correlation coefficients between plant parameters and soil biochemical properties (n = 56)
Plant parameters |
OC |
NH4+-N |
AP |
Soil respiration |
Microbial biomass |
Soil enzymes |
DTPA-extractable metals |
Dehydrogenase |
β-Glucosidase |
Urease |
Phosphatase |
Cd |
Cu |
Pb |
Zn |
*p < 0.05, **p < 0.01 |
Cover |
0.916** |
0.922** |
0.944** |
0.847** |
0.707** |
0.944** |
0.847** |
0.948** |
0.883** |
−0.799** |
−0.905** |
−0.745** |
−0.605** |
Biomass |
0.830** |
0.928** |
0.926** |
0.839** |
0.653** |
0.948** |
0.858** |
0.849** |
0.866** |
−0.719** |
−0.893** |
−0.670** |
−0.633** |
Cd |
−0.795** |
−0.845** |
−0.840** |
−0.820** |
−0.741** |
−0.870** |
−0.784** |
−0.835** |
−0.802** |
0.706** |
0.845** |
0.543* |
0.631** |
Cu |
−0.779** |
−0.789** |
−0.786** |
−0.719** |
−0.596** |
−0.808** |
−0.628** |
−0.776** |
−0.689** |
0.806** |
0.775** |
0.806** |
0.476* |
Pb |
−0.842** |
−0.872** |
−0.875** |
−0.847** |
−0.699** |
−0.855** |
−0.806** |
−0.883** |
−0.785** |
0.645** |
0.812** |
0.654** |
0.484* |
Zn |
−0.823** |
−0.867** |
−0.858** |
−0.814** |
−0.677** |
−0.863** |
−0.772** |
−0.867** |
−0.799** |
0.708** |
0.861** |
0.546* |
0.486* |
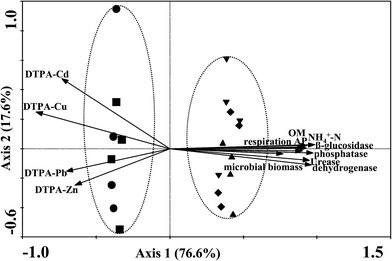 |
| Fig. 6 Ordination biplot of the canonical correspondence analysis (n = 380). Symbols: tailings (●), NTS (■), SSV (▲), MHR (▼), SMC (◆). | |
4 Discussion
Phytostabilization is recognized as a potentially cost-effective, ecologically sound and sustainable solution for the remediation of heavy metal-contaminated soils and mine tailings.2 The success of phytostabilization on the mine tailings mainly depends on the improvement of the substrate. Organic amendment is a major requirement which can provide essential nutrients, rebuild soil structure, re-establish microbial populations, and eventually allow plant establishment and subsequent vegetation development.25
As other mine tailings,2,26,27 heavy metal toxicity is the major constraint for ecological restoration of this Pb/Zn mine tailings. In the present field trial, the application of industrial by-products (SSV, MHR and SMC) as organic amendments significantly reduced DTPA-extractable Cd, Cu, Pb and Zn compared to the control tailings (Fig. 1), which was consistent with the results of our earlier pot study.10 DTPA-extractable metal concentrations usually well predict the metal fraction readily available to plants and microorganisms (i.e. metal bioavailability) and this reduction in DTPA-extractable metal concentrations may be attributed to the immobilization processes by the industrial by-product amendments. The main mechanisms by which they immobilize metals are probably based on adsorption, complexation and/or precipitation reactions.4 It has been reported that SMC has a very high sorption capacity for Cd, Pb and Cr owing to the presence of hydroxyl, phosphoryl and phenolic functional groups on its surface.28 In addition, no significant changes were found in DTPA-extractable Cd, Cu, Pb and Zn concentrations within the one-year remediation period. Similar results were also reported by other authors,29,30 which can be related to the plant rhizospheric processes since root exudates and microbial activity can increase the solubility of metal ions in the rhizosphere.
Apart from reducing metal bioavailability, another important aim of phytostabilization is to restore the ecological function and health of mine tailings. As expected, the addition of SSV, MHR and SMC led to significant increase in soil nutrient elements such as OC, NH4+-N and AP (Fig. 2). This improvement of the tailings conditions with the application of these amendments allowed microbial community development and facilitated soil-forming processes.1,31 In recent years, microbial parameters have increasingly been used as indicators of soil quality to evaluate the success of remediation efforts.32,33 In the present study, the addition of SSV, MHR and SMC significantly enhanced soil respiration, microbial biomass and soil enzyme activities when compared to the controls (Fig. 3 and 4). This is in accordance with the findings of Kumpiene et al.34 who found that phytostabilization significantly increased soil respiration, microbial biomass and the activity of key soil enzymes, indicating a clear enhancement of soil function. In addition, we also observed a slightly increasing trend in nutritional status (OC, NH4+-N and AP) and microbial parameters (microbial biomass and enzyme activities) in SSV, MHR and SMC treatments (Fig. 2–4). This result supported the conclusion that the addition of organic amendments led to a larger and more active microflora and nutrient accumulation in the mine tailings.10,34
Beneficial effects of the three industrial by-products were also observed in vegetation characteristics, as reflected by the vegetation cover, biomass and heavy metal accumulation in plant tissues (Fig. 5 and Table 1). In the present field trial, the application of these amendments promoted seed germination, seedling growth, and subsequently increased the vegetation cover and biomass on SSV, MHR and SMC subplots (Fig. 5). Plant growth may improve soil nutrient accumulation and microbiological function, which was evidenced by the significant positive correlations between the vegetation cover and biomass and soil nutrient status (OC, NH4+-N and AP) and microbial parameters (soil respiration, microbial biomass and enzyme activities) (Table 2). Another particular concern associated with the revegetation of mine tailings is the accumulation of heavy metals in the above-ground parts of plants. From the viewpoint of stabilizing metals in the mine tailings, the desirable species should always absorb or transport as low as possible heavy metals from the tailings, thereby limiting the propagation of metals into the food chain.2,3 Moreover, the advantages of using native plant species are generally considerable since they are ecologically adapted to the local environmental conditions.35 The species selected (L. perenne, C. dactylon, M. sativa and D. indicum) for this study are native and have been reported as metallophytes, which have all been widely employed as pioneer species for phytostabilization of metal-contaminated soils.5,7,8 Almost all concentrations of Cd, Cu, Pb and Zn in above-ground parts of the plants were below toxicity limits of the US toxicity limits for cattle (Cd ≤ 10 mg kg−1, Cu ≤ 40 mg kg−1, Pb ≤ 100 mg kg−1 and Zn ≤ 500 mg kg−1),36 and the addition of SSV, MHR and SMC significantly decreased the shoot metal concentrations (Cd, Cu, Pb and Zn) of L. perenne and C. dactylon compared to the tailings and NTS treatments (Table 1). This was consistent with the results of significant negative correlation between the metal concentrations in plants and soil nutrient elements and microbial parameters (Table 2). However, we did not ascertain how much of the improvement in the tailings conditions was due to the addition of organic amendments and how much of it was due to the plant development. The strong correlation between plant parameters and soil biochemical properties (Fig. 6) demonstrated that these factors are likely to be synergistic in phytostabilization of this Pb/Zn mine tailings.
5 Conclusions
The results obtained from the present field experiment indicate that phytostabilization (plants together with the application of amendments) can be a promising strategy for the restoration of mine tailings. The three industrial by-products (SSV, MHR and SMC) were equally effective in reducing the levels of bioavailable metals in the mine tailings, increasing soil nutrient status (organic C, ammonium-N and available P) and enhancing soil respiration, microbial biomass and enzyme activities. The addition of SSV, MHR and SMC promoted plant growth (vegetation cover and biomass) and decreased heavy metal uptake and accumulation in harvestable plant tissues. Although caution must be used when extrapolating from controlled laboratory experiments to field conditions, our work showed similar results in both the pot and field trials.
Acknowledgements
This work was supported by the National Natural Science Foundation of China (Nos. 41561076, 41471257 and 21266008), Postdoctoral Science Foundation of Central South University (No. 131424) and “Environment and Energy Materials and Deep Processing of Mineral Resources in Wuling Mountain” for Science and Technology Innovative Research Team in Higher Educational Institutions of Hunan Province. Particularly, we thank Professor AJM Baker (Universities of Melbourne and Queensland, Australia, and Sheffield, UK) for his help in the improvement of this paper.
References
- S. Kabas, A. Faz, J. A. Acosta, R. Zornoza, S. Martinez-Martínez, D. M. Carmona and J. Bech, J. Geochem. Explor., 2012, 123, 69 CrossRef CAS.
- S. H. Lee, W. H. Ji, W. S. Lee, N. Koo, I. H. Koh, M. S. Kim and J. S. Park, J. Environ. Manage., 2014, 139, 15 CrossRef CAS PubMed.
- G. M. Tordoff, A. J. M. Baker and A. J. Willis, Chemosphere, 2000, 41, 219 CrossRef CAS PubMed.
- M. A. Galende, J. M. Becerril, M. T. Gómez-Sagasti, O. Barrutia, L. Epelde, C. Garbisu and A. Hernández, Water, Air, Soil Pollut., 2014, 225, 1863 CrossRef.
- M. O. Mendez and R. M. Maier, Environ. Health Perspect., 2008, 116, 278 CrossRef CAS PubMed.
- X. F. Li and L. B. Huang, Crit. Rev. Environ. Sci. Technol., 2015, 45, 813 CrossRef CAS.
- P. Alvarenga, A. P. Gonçalves, R. M. Fernandes, A. de Varennes, G. Vallini, E. Duarte and A. C. Cunha-Queda, Chemosphere, 2009, 74, 1292 CrossRef CAS PubMed.
- P. Alvarenga, P. Palma, A. P. Gonçalves, R. M. Fernandes, A. de Varennes, G. Vallini, E. Duarte and A. C. Cunha-Queda, Chemosphere, 2009, 74, 1301 CrossRef CAS PubMed.
- K. K. Chiu, Z. H. Ye and M. H. Wong, Bioresour. Technol., 2006, 97, 158 CrossRef CAS PubMed.
- S. X. Yang, J. B. Cao, W. Y. Hu, X. J. Zhang and C. Dun, Environ. Sci.: Processes Impacts, 2013, 15, 2059 CAS.
- M. S. Li, Sci. Total Environ., 2006, 357, 38 CrossRef CAS PubMed.
- T. Pardo, R. Clemente, P. Alvarenga and M. P. Bernal, Chemosphere, 2014, 107, 101 CrossRef CAS PubMed.
-
D. W. Nelson and L. E. Sommers, in Agronomy Monograph, ed. A. L. Page, American Society of Agronomy and Soil Science Society of America, Madison, USA, 1982, vol. 9, p. 595 Search PubMed.
-
D. G. Maynard, Y. P. Kalra and J. A. Crumbaugh, Nitrate and Exchangeable Ammonium Nitrogen in Soil Sampling and Methods of Analysis, CRC Press, USA, 2008, p. 71 Search PubMed.
- R. H. Bray and L. T. Kurtz, Soil Sci., 1945, 59, 39 CrossRef CAS.
- W. L. Lindsay and W. A. Norvell, Soil Sci. Soc. Am. J., 1978, 42, 421 CrossRef CAS.
-
J. P. E. Anderson, Soil respiration, in Methods of Soil Analysis, Soil Science Society of America, Madison, Wisconsin, 1982, p. 837 Search PubMed.
- E. D. Vance, P. C. Brookes and D. S. Jenkinson, Soil Biol. Biochem., 1987, 19, 703 CrossRef CAS.
- A. Thalmann, Landwirtsch. Forsch., 1968, 21, 249 CAS.
- G. Hoffmann and M. Dedeken, Z. Pflanzenernaehr. Bodenkd., 1966, 108, 195 Search PubMed.
- E. Kandeler and H. Gerber, Biol. Fertil. Soils, 1988, 6, 68 CrossRef CAS.
- A. M. Tabatabai and M. J. Bremner, Soil Biol. Biochem., 1969, 1, 301 CrossRef.
-
S. E. Allen, Chemical Analysis of Ecological Materials, Blackwell Scientific Press, Oxford, 1989 Search PubMed.
-
C. J. F. Ter Braak and P. Šamilauer, CANOCO Reference Manual and CanoDraw for Windows User's Guide: Software for Canonical Community Ordination (Version 4.5), Microcomputer Power, Ithaca, USA, 2002 Search PubMed.
- C. Santibañez, L. M. de la Fuente, E. Bustamante, S. Silva, P. León-Lobos and R. Ginocchio, Appl. Environ. Soil Sci., 2012, 1, 1 CrossRef.
- X. Wang, Y. G. Liu, G. M. Zeng, L. Y. Chai, X. Xiao, X. C. Song and Z. Y. Min, Chemosphere, 2008, 72, 1260 CrossRef CAS PubMed.
- Y. M. Zhu, C. Y. Wei and L. S. Yang, Acta Ecol. Sin., 2010, 30, 178 CrossRef.
- S. N. Jordan, G. J. Mullen and R. G. Courtney, Bioresour. Technol., 2008, 99, 8125 CrossRef CAS PubMed.
- S. X. Yang, B. Liao, J. T. Li, T. Guo and W. S. Shu, Chemosphere, 2010, 80, 852 CrossRef CAS PubMed.
- C. B. Zhang, L. N. Hang, T. G. Luan, J. Jin and C. Y. Lan, Geoderma, 2006, 136, 555 CrossRef CAS.
- J. J. Li, X. M. Zhou, J. X. Yan, H. J. Li and J. Z. He, Appl. Soil Ecol., 2015, 87, 56 CrossRef.
- K. Ciarkowska, K. Sołek-Podwika and J. Wieczorek, J. Environ. Manage., 2014, 132, 250 CrossRef CAS PubMed.
- H. Schimann, C. Petit-Jean, S. Guitet, T. Reis, A. M. Domenach and J. C. Roggy, Ecol. Indic., 2012, 20, 34 CrossRef CAS.
- J. Kumpiene, G. Guerri, L. Landi, G. Pietramellara, P. Nannipieri and G. Renella, Ecotoxicol. Environ. Saf., 2009, 72, 115 CrossRef CAS PubMed.
- G. Bacchetta, G. Cappai, A. Carucci and E. Tamburini, Bull. Environ. Contam. Toxicol., 2015, 94, 326 CrossRef CAS PubMed.
-
NRC (National Research Council), Mineral Tolerance of Animals, National Academies Press, Washington, 2005 Search PubMed.
Footnote |
† Electronic supplementary information (ESI) available. See DOI: 10.1039/c5em00471c |
|
This journal is © The Royal Society of Chemistry 2016 |
Click here to see how this site uses Cookies. View our privacy policy here.