DOI:
10.1039/C6LC90088G
(Focus)
Lab Chip, 2016,
16, 3626-3630
Miniaturized soft bio-hybrid robotics: a step forward into healthcare applications
First published on 23rd August 2016
Abstract
Soft robotics is an emerging discipline that employs soft flexible materials such as fluids, gels and elastomers in order to enhance the use of robotics in healthcare applications. Compared to their rigid counterparts, soft robotic systems have flexible and rheological properties that are closely related to biological systems, thus allowing the development of adaptive and flexible interactions with complex dynamic environments. With new technologies arising in bioengineering, the integration of living cells into soft robotic systems offers the possibility of accomplishing multiple complex functions such as sensing and actuating upon external stimuli. These emerging bio-hybrid systems are showing promising outcomes and opening up new avenues in the field of soft robotics for applications in healthcare and other fields.
Introduction
The evolution of biological systems has resulted in sophisticated mechanisms able to sense and actuate as a response to external stimuli as well as to adapt, self-repair and self-assemble. Newly fabricated robotic devices may significantly benefit from these unique properties to enhance their suitability and performance in healthcare related applications, including diagnosis, drug therapy, and surgery. However, in artificial systems, the achievement of such functionalities still remains a challenge. In this sense, the rigidity of materials used in conventional robotics can limit their performance in healthcare-related applications. As an alternative, soft robotics is emerging as a new discipline aiming to overcome the limitations of conventional robotic devices through exploring soft matter (i.e. fluids, gels, soft polymers and deformable materials) and biologically inspired engineering.1
So far, the use of bio-inspired smart materials and the integration of living cells into artificial micro-scaled systems have shown promising outcomes for the accomplishment of multiple and complex functions such as sensing, processing and responding to dynamic environmental stimuli in a very controlled manner.2 Moreover, these systems are biocompatible and can be operated through non-invasive control mechanisms such as electrical, chemical or optical stimulation, without the need for large-scale external driving systems or toxic fuels, which is crucial for biomedical applications.3
In this Focus article, we will discuss some of the recent advances in bio-inspired materials and bio-hybrid systems for soft robotics applications in biomedicine.
Bio-inspired materials for soft robotics
One of the main challenges in the field of robotics with regard to its applicability in medicine is the rigidity of the materials used. The lack of mechanical compliance between these materials and biological systems accounts for their low biocompatibility, poor capability for multifunctionality and low elasticity.1 Material rigidity can be measured by the Young's modulus, which defines the relationship between stress (force per unit area) and strain (proportional deformation) in a material. Thus, it can be very useful for measuring the elasticity of materials for soft robotics.4Fig. 1A shows a comparison between different materials, either artificial or biological, regarding their Young's modulus. Some of the most commonly used materials for soft robotics are polymers, especially elastomers, since they can provide a matching compliance with the properties of biological tissue in terms of elasticity and flexibility. Elastomeric soft robots can be actuated by applying pressure to deform their structure, what is known as pneumatic artificial muscles (PAMs) or McKibben actuators.5 In this regard, Martinez et al. developed flexible soft robotic tentacles consisting of elastomeric materials, which were fabricated using soft lithography (Fig. 1B). Upon pressurization, the tentacles showed a complex three dimensional motion.6
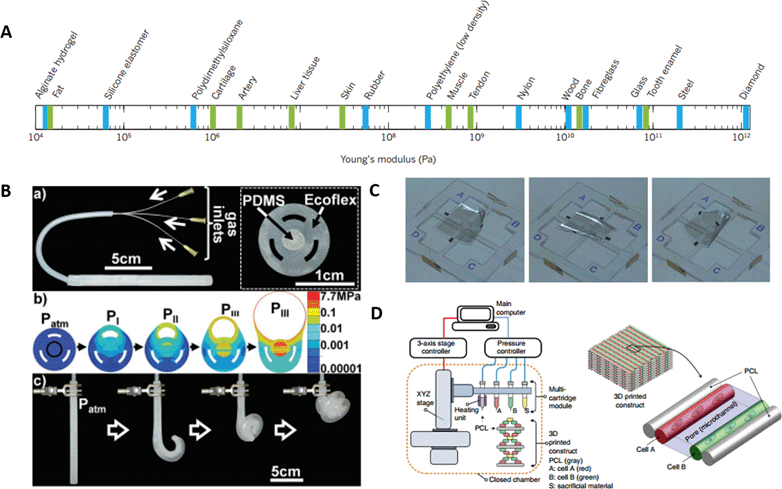 |
| Fig. 1 Elastic properties of the most commonly used materials for soft robotics and examples of their applications. A) Young's modulus of the most commonly used materials in soft robotic applications (blue) and of biological tissues (green). Reprinted with permission from ref. 4. B) Elastomeric based tentacle that is able to perform a complex 3D motion upon pressurization of a micropneumatic channel. Reprinted with permission from ref. 6. C) Multi-layered dielectric elastomer based actuator combined with carbon nanotube electrodes for low-voltage input actuation. Reprinted with permission from ref. 8. D) Schematic representation of a 3D-bioprinting system which is able to create structures that combine stimuli-responsive polymers, hydrogels and living cells. Reprinted with permission from ref. 10. | |
Alternatively, other groups have focused their research on the development of electrically activated soft actuators based on electroactive polymers (EAPs), which can be divided into electronic EAPs and ionic EAPs. Among these, dielectric elastomers have attracted a great deal of attention for the development of muscle-like actuators due to their capability to convert electrical energy into mechanical energy in a reversible manner.7 Recently, Duduta et al. have developed a new dielectric-elastomer-based actuator which combines the elastomeric material with carbon nanotube electrodes in order to achieve a high performance actuator system without the need for pre-stretching and with low voltage input (Fig. 1C), which are the main limitations for these types of actuators.8 Apart from the elastic properties of materials, when fabricating soft robotics for medical applications their biocompatibility must be taken into account. So far, a wide range of biocompatible and biodegradable synthetic polymers have been developed.9 In addition, recent advances in 3D bioprinting techniques are opening up new ventures in the field of tissue engineering by the creation of combined structures using stimulus-responsive polymers and hydrogel–cell mixtures as shown in Fig. 1D.10 These novel techniques could significantly benefit the creation of bio-hybrid systems in order to achieve full biocompatibility and compliance in soft robotics.
Cell-powered bio-hybrid soft robots
Actuation in animals is mainly accomplished by muscle cells, which respond to an electrical stimulus generating contractile forces. Therefore, contractile cells have been widely explored for the creation of bio-hybrid actuator microsystems based on micropillars, cantilevers and thin films.2 Moreover, it has been recently described that certain designs of bio-hybrid devices can lead to untethered directional locomotion, which can be highly repeatable, adaptable and controllable. Such devices are known as “bio-bots” and will be described in this section.
Cardiac cell powered micro-robots
The spontaneous self-beating properties of cardiomyocytes have made them very attractive candidates for the development of cell-based actuators. Fig. 2A shows the formation of a bio-bot composed by self-organizing cardiomyocytes in 2D sheets onto a 3D printed hydrogel cantilever structure.11 The tension generated by cardiomyocyte beating results in curvature of the sheet. An untethered walking motion of the bio-bot is achieved when asymmetry is introduced into the structure (Fig. 2A, left). Recently, a more sophisticated bio-hybrid system incorporating an external remote control through light has been developed by Park et al.12 In their work, the authors have fabricated a light-responsive stingray analogue by combining tissue engineering and soft materials with optogenetics. This allows a highly precise and controllable locomotion guided by the response of the integrated sensory-motor system to external light stimuli (Fig. 2C). In order for this system to be built, a three-dimensional polydimethylsiloxane (PDMS) body was fabricated using a titanium mold. Then, a chemically neutral skeleton was created through thermal gold evaporation, which was followed by the spin-coating of a PDMS interstitial layer. Finally, a layer of serpentine-like aligned cardiomyocytes was created by fibronectin microcontact printing. The combination of different body rigidities along the anterior–posterior axis and different flexibility of the cardiomyocyte fins along the proximal–distal axis creates an asymmetry that allows forward motion upon cardiomyocyte contractions (Fig. 2C).
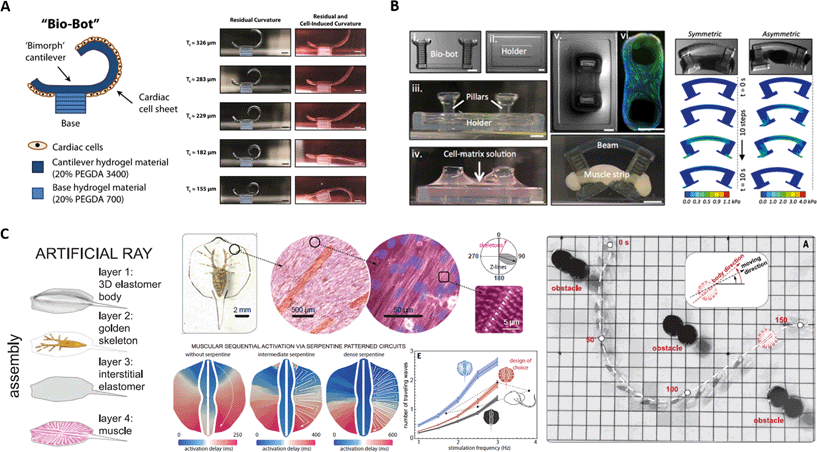 |
| Fig. 2 Bio-hybrid soft robots powered by muscle cells. A) Bio-bot composed of 2D cardiomyocyte sheets on a 3D-printed hydrogel cantilever. Reprinted with permission from ref. 11. B) Skeletal-muscle cell powered micro-walker. Reprinted with permission from ref. 13. C) Artificial ray powered by cardiomyocytes controlled by optical external stimuli. Reprinted with permission from ref. 12. | |
Skeletal muscle cell powered micro-robots
Unlike cardiomyocytes, skeletal muscle cells do not contract spontaneously, thus allowing a better control over their actuation.13 In addition, skeletal muscle cells are primarily responsible for actuation in animals, where they organize following a modular tissue architecture which produces uniaxial force that ultimately generates motion. Thus, this cell type holds great potential for the fabrication of bio-hybrid robotic systems. Cvetkovic et al. reported the fabrication of a bio-bot through the integration of an engineered muscle into a 3D printed polyethyleneglycol-diacrylate (PEG-DA) flexible scaffold consisting of two pillars connected by a beam (Fig. 2B).13 This work was previously highlighted in another Focus article.14 The myotube contraction would create forces able to tense the flexible skeleton upon the application of an external electrical stimulus. Furthermore, the asymmetric skeleton structure generated a directional locomotion due to asymmetric pillar displacements by the non-uniform distribution of stress in the hydrogel skeleton (Fig. 2B). Recently, the same group has reported that skeletal muscle cell-based bio-bots can be further engineered by the addition of optical control mechanisms and the shape of myotubes. Besides, not only did the bio-bot show a highly precise control over directional locomotion but also a dynamic adaptation to the environment through “exercise” training stimuli.15
External control of bio-hybrid robots
Control mechanisms for hybrid bio-robots are necessary for the effective actuation of these devices. Fig. 3 displays several examples of the most commonly found control methods in the literature – electrical, chemical and optical. Electrical control of hybrid micro-devices based on muscle cells tries to emulate the action potentials generated by neurons that control the contraction of skeletal muscle cells in animals. Fig. 3B shows an example of a setup for electrical stimulation of myotube strips in a PEDOT-coated microelectrode array.16 Another approach can be placing Pt electrodes on two sides of a culture dish containing the sample with an electrical stimulation medium, as used by Cvetkovic et al.13 With a waveform generator, several pulses of different frequencies can be applied in order to stimulate the contraction of myotubes. This method allows a precise control of the contraction frequency, equal to the pulse frequency.
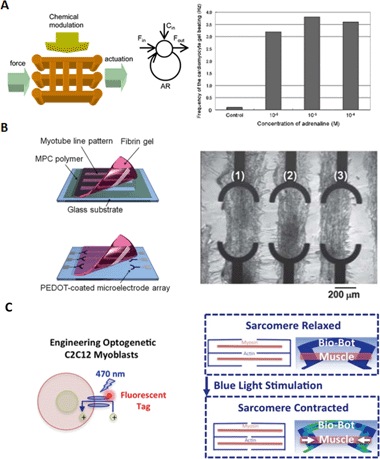 |
| Fig. 3 Schematic representation of the three main control mechanisms of hybrid bio-bots: chemical (A), electrical (B) and optical (C). A) Left: Cardiac muscle network with chemical stimulation and feedback loop. Right: Frequency of contraction with respect to the concentration of adrenaline. Reprinted with permission from ref. 17. B) Myotube lines patterned in a fibrin gel transferred to a PEDOT-coated microelectrode array for their electrical stimulation. Reprinted with permission from ref. 16. C) Left: Schematic representation of C2C12 myoblasts transduced with fluorescent channelrhodopsin-2, which can be activated with blue light. Right: schematic representation of bio-bots contraction upon light stimulation. Reprinted with permission from ref. 15. | |
On the other hand, chemical and mechanical cues from the environment can have important effects on the behaviour of cardiac and skeletal tissue. The understanding of these effects is especially relevant for the fabrication and control of hybrid bio-robots. The creation of cardiomyocyte collagen networks, such as the one shown in Fig. 3A, can help in assessing the implications of mechanical stress. Forces exerted during contraction, as well as those generated by chemical stimuli, propagate through the network in a feedback loop. Modulation of the network characteristics and the presence of chemical stimuli can help control the self-beating capabilities of the cardiac tissue. Hoshino et al. studied these effects for different kinds of gel networks and the addition of adrenaline (Fig. 3A, right).17 They proved that the presence of adrenaline enhanced the depolarization speed, increasing the wave velocity and the frequency of contraction.
The genetic modification of biological tissues can provide more complex features to the bio-bots, such as light sensitive actuation, in what is known as optogenetics. Raman et al. developed a skeletal-muscle bio-bot that could be activated through optical stimulation.15 For this, they transduced a blue-light sensitive channel, channelrhodopsin-2 (ChR2), that could activate the contraction of C2C12 skeletal muscle cells upon light exposure (Fig. 3C). The same kind of genetic modification was adopted by Park et al. in their soft-robotic hybrid stingray.12 However, in the latter, the aim was not only contractile control, but also the maneuvering of the bio-bot by taking advantage of this feature, generating phototaxis. The cardiac muscle fins of the stingray were patterned in a serpentine fashion, adding physical constraints to the propagation of contractions, as in a muscle circuit. Light stimulus at the front part of the ray induced the propagation of an action potential, causing forward movement (Fig. 2C, right). Modulation of the pulse frequency at the right or left part of the frontal fins allowed right and left maneuverability, since the action potentials were spatially and temporally modulated by the serpentine pattern of the bio-robot.
The contractile forces generated by bio-bots can not only be controlled, but also modulated according to specific needs. This is the case in the study carried out by Chan et al., which proved that the amount of 2D-printed muscle strips can be used to modulate the total force generated during contraction of the 3D tissue, while keeping constant contraction and relaxation times.18 The shape of the muscle tissue, and not only the number of strips, plays a crucial role in the response to control mechanisms. Raman et al. checked the difference between ring- and strip-shaped skeletal muscle tissues after they were externally activated using blue light and electrical stimuli.15 Optical stimulation in muscle strips was found to be clearly hindered with respect to muscle rings, while electrical stimulation was the same in both cases. This difference was attributed to the higher myotube density in muscle ring actuators. With this architecture, light stimulation could excite a larger amount of myotubes, resulting in greater contraction, when compared to muscle strip actuators.
Other methods such as magnetic or temperature control of muscle bio-bots can also be envisaged. Furthermore, ultrasonics could also become an important mechanism of bio-robot control, since it has been proven to modulate cytoskeleton contractile forces via the excitation of lipid microbubbles.19
Conclusions and outlook
Soft robotic systems based on bio-inspired materials and the hybrid integration of biological cells have gained increasing attention over the past years, since they offer a promising new approach for combining the best features of artificial and biological systems. The achievements presented in this Focus article demonstrate that such hybrid integration is possible. Advances in this field, especially since the appearance of 3D printing and bio-printing, have opened a path toward new actuators, sensors, and mobile systems with unprecedented sensitivity and controlled actuation capabilities. Hybrid bio-bots could surpass the performance of artificial robotic systems, especially in medical applications, and have a tremendous impact in the field. There are, however, certain challenges that will need to be overcome, such as the lifetime of these devices and the supply of power sources, which could be solved with the addition of more complex functionalities like chemical supply systems based on bio-mimetic vessels.
Acknowledgements
The authors thank funding from the European Research Council under the European Union's Seventh Framework Program (FP7/20072013)/ERC grant agreement no. 311529 (LT-NRBS) and the Spanish MINECO under grants CTQ2015-68879-R (MICRODIA) and CTQ2015-72471-EXP (Enzwim). T. P. thanks MINECO for the Juan de la Cierva fellowship.
References
- C. Majidi, Soft Robot., 2014, 1, 5–11 CrossRef.
- R. W. Carlsen and M. Sitti, Small, 2014, 10, 3831–3851 CrossRef CAS PubMed.
- S. Sánchez, L. Soler and J. Katuri, Angew. Chem., Int. Ed., 2015, 54, 1414–1444 CrossRef PubMed.
- D. Rus and M. T. Tolley, Nature, 2015, 521, 467–475 CrossRef CAS PubMed.
- C.-P. Chou and B. Hannaford, IEEE Trans. Rob. Autom., 1996, 12, 90–102 CrossRef.
- R. V. Martinez, J. L. Branch, C. R. Fish, L. Jin, R. F. Shepherd, R. M. D. Nunes, Z. Suo and G. M. Whitesides, Adv. Mater., 2013, 25, 205–212 CrossRef CAS PubMed.
- L. J. Romasanta, M. A. Lopez-Manchado and R. Verdejo, Prog. Polym. Sci., 2015, 51, 188–211 CrossRef CAS.
- M. Duduta, R. J. Wood and D. R. Clarke, Adv. Mater., 2016 DOI:10.1002/adma.201601842.
- M. Tanaka, K. Sato, E. Kitakami, S. Kobayashi, T. Hoshiba and K. Fukushima, Polym. J., 2015, 47, 114–121 CrossRef CAS.
- H. Kang, S. J. Lee, I. K. Ko, C. Kengla, J. J. Yoo and A. Atala, Nat. Biotechnol., 2016, 34, 312–319 CrossRef CAS PubMed.
- V. Chan, K. Park, M. B. Collens, H. Kong, T. A. Saif and R. Bashir, Sci. Rep., 2012, 2, 857 Search PubMed.
- S.-J. Park, M. Gazzola, K. S. Park, S. Park, V. Di Santo, E. L. Blevins, J. U. Lind, P. H. Campbell, S. Dauth and A. K. Capulli,
et al.
, Science, 2016, 353, 158–162 CrossRef CAS PubMed.
- C. Cvetkovic, R. Raman, V. Chan, B. J. Williams, M. Tolish, P. Bajaj, M. S. Sakar, H. H. Asada, M. T. A. Saif and R. Bashir, Proc. Natl. Acad. Sci. U. S. A., 2014, 111, 10125–10130 CrossRef CAS PubMed.
- M. M. Stanton, C. Trichet-Paredes and S. Sánchez, Lab Chip, 2015, 15, 1634–1637 RSC.
- R. Raman, C. Cvetkovic, S. G. M. Uzel, R. J. Platt, P. Sengupta, R. D. Kamm and R. Bashir, Proc. Natl. Acad. Sci. U. S. A., 2016, 113, 3497–3502 CrossRef CAS PubMed.
- K. Nagamine, T. Kawashima, S. Sekine, Y. Ido, M. Kanzaki and M. Nishizawa, Lab Chip, 2011, 11, 513–517 RSC.
- T. Hoshino, K. Imagawa, Y. Akiyama and K. Morishima, Biomed. Microdevices, 2012, 14, 969–977 CrossRef CAS PubMed.
- V. Chan, D. M. Neal, S. G. M. Uzel, H. Kim, R. Bashir and H. H. Asada, Lab Chip, 2015, 15, 2258–2268 RSC.
- Z. Fan, Y. Sun, Di Chen, D. Tay, W. Chen, C. X. Deng and J. Fu, Sci. Rep., 2013, 3, 2176 Search PubMed.
|
This journal is © The Royal Society of Chemistry 2016 |
Click here to see how this site uses Cookies. View our privacy policy here.