DOI:
10.1039/C5NJ01701G
(Paper)
New J. Chem., 2016,
40, 423-433
In-vitro evaluation of layered double hydroxide–Fe3O4 magnetic nanohybrids for thermo-chemotherapy†
Received
(in Montpellier, France)
1st July 2015
, Accepted 29th October 2015
First published on 4th November 2015
Abstract
Among two-dimensional nanomaterials, layered double hydroxides (LDHs) are of great interest in biomedical applications due to their unique properties and layered structure. Superparamagnetic iron oxide nanoparticles (Fe3O4) are also well known for their tailorable properties, high magnetization values and biocompatibility. The objectives of our current work are to combine LDHs with magnetic nanoparticles in order to widen the horizons of their applications in cancer therapy. This work undertakes a facile chemical approach for the fabrication of Fe3O4-conjugated Mg–Al layered double hydroxide magnetic nanohybrids (MNHs). The successful fabrication of these MNHs was evident from X-ray diffraction analysis, infrared spectroscopy, X-ray photoelectron spectroscopy, and zeta potential measurements. These MNHs were explored as possible heating platforms for magnetic hyperthermia as well as drug-delivery vectors to cancer cells. A high degree of drug-loading efficiency (∼99%) for doxorubicin (Dox), with ∼90% release in high proton environments was observed. In addition, the nature of the host–drug interactions was systematically investigated by fluorescence spectroscopy. These MNHs were seen to be biocompatible with murine fibroblast (L929) and human cervical (HeLa) cell lines. To exemplify the therapeutic performances of Dox-loaded MNHs, the IC50 (50% inhibitory concentration) value was also evaluated against HeLa cells. Calorimetric measurements revealed the specific absorption rates of 98.4 and 73.5 W g−1 for Fe3O4 and MNHs, respectively. In addition, the MNHs acted as a “cut-off switch” to maintain the hyperthermic temperature. As hyperthermia agents, these MNHs showed that a 20 min exposure to an alternating current magnetic field (ACMF) is adequate to inhibit the proliferation of HeLa cells and decrease the cell population significantly. In conclusion, the results established that these MNHs open up avenues of much more effective anticancer therapy.
Introduction
The current scenario of cancer treatment in clinical settings mainly includes radiotherapy and chemotherapy. Radiotherapy uses high-energy radiation to damage, sensitize or induce cell-cycle arrest in the cancer cells.1 The type and quantity of radiation selected are largely based on the patient's medical condition, tumour size and region and, thus, are very carefully calculated. Classical chemotherapy in the treatment of cancer involves the use of a variety of chemicals singularly or in multitudes, in order to kill the tumour cells.2,3 However, both of these therapeutic approaches have generic effects, and affect the normal cells in proportions similar to cancer cells. These side effects necessitate a therapeutic alternative that can differentiate between normal and cancer cells and act selectively on the targeted tissue. Thus, the field of thermotherapy is being investigated as an alternative cure in the past few decades for cancer. Recent years have marked the development of a variety of delivery systems for various applications in therapeutics or diagnostics or both.4 Among a vast variety of nanocarriers, superparamagnetic nanoparticles have been widely used in different biomedical applications such as drug delivery, bio-separation, immunoassay, magnetic resonance imaging and magnetic hyperthermia.5–7
An interesting property exhibited by these superparamagnetic nanoparticles is their ability to heat up on exposure to an ACMF. The heat generated by superparamagnetic nanoparticles is due to either the rotation of magnetic spins within the particle (Néel relaxation) or the rotation of the particle as a whole (Brownian relaxation).8 The heat dissipated during these relaxation processes is given by eqn (1):
where
χ′′ is the imaginary part of susceptibility,
ν and
H are the frequency and the amplitude of the applied ACMF, respectively. The specific absorption rate (SAR) is the primary parameter that determines the heating capability of these nanoparticles, and is proportional to the rate of temperature increase.
8 This dissipated heat has been utilized in the treatment of cancer; thus, these nanoparticles are capable of performing the role of anticancer agents by themselves.
9 The interest in using superparamagnetic nanoparticles has also increased because of a certain degree of possibility to be targeted to the tumour site using an external magnet (magnetic drug targeting), thereby reducing the accumulation of nanoparticles at undesired sites, as seen in other classical therapies such as chemotherapy and radiotherapy.
10 Also, high biocompatibility, biodegradability, and non-immunogenicity set these nanoparticles apart for their usefulness in magnetic hyperthermia.
10,11
However, the continuous exposure of these nanoparticles to an ACMF might lead to uncontrolled and unwanted heating, and could extend beyond the tumour boundaries, becoming detrimental to neighbouring normal tissues as well.12 To meet this challenge, we require a material that can sense the unwanted heating and cut-off after reaching a threshold temperature, not allowing the material to heat beyond this threshold temperature. While dealing with cancer cells, this threshold temperature window exists between 42 and 45 °C. A material possessing the required properties of this “cut-off switch” should have customisable architectural, chemical and physical properties, biocompatibility, biodegradability, and the ability to not generate any immunological response when present in a biological system. LDHs were thus explored as this “cut-off switch” in combination with magnetite as a hyperthermic entity.
LDHs are hydrotalcite-like compounds that have brucite-like positively charged layers that are balanced by counter-anions and water molecules; their general formula is [M2+1−xM3+x(OH)2][An−x/n·mH2O]. M2+ and M3+ are metal cations incorporated into positive layers and An− are the balancing anions present in the interlayer spacing. The high anionic exchange capability, the high surface charge (readily available for binding interactions), and the biocompatible and non-immunogenic nature of LDHs have made them a prime choice as platforms in biomedical applications.13–15 Also, LDHs and their nanohybrids have been used for various applications such as controlled drug and gene delivery materials, catalysts, electrode materials, switchable magnetic hybrids and many more.16–19 With regard to the toxicity of LDHs containing Al in their layers, these are less toxic to both normal and cancer cells as compared to other inorganic materials like silica, and carbon nanotubes. As observed and reported by Choy et al., Mg–Al LDH shows biocompatible behaviour towards many cell lines like A549, L132, HeLa and HOS.20 These 2D nanomaterials have also been the choice for delivering a variety of drugs and nucleotide molecules establishing their biocompatibility.21,22
These unique physico-chemical properties, high biocompatibility, biodegradability and low-immunogenicity make Fe3O4 nanoparticles (magnetic) and 2-dimensional LDH (non-magnetic) stand out as potential candidates for biomedical applications. Thus, combining these nanoplatforms might generate the required properties in addressing the challenge in the uncontrolled heating in magnetic hyperthermia. Thus, the current work demonstrates the synthesis of a hybrid material and describes the potential of LDH as the “cut-off switch”, which when combined with Fe3O4, does not allow the temperature to rise beyond our required window.23
Experimental
Synthesis of nanoparticles and magnetic nanohybrids
Mg–Al LDH was synthesized using a method described in previous literature, with minor modifications.24 In brief, an aqueous solution of Mg2+ and Al3+ (molar ratio 3
:
1) was added to 0.3 M aqueous NaOH solution and stirred for 10–15 min. The white precipitate obtained was then centrifuged and washed with MilliQ water 2–3 times. The precipitate was then re-dispersed in water and treated hydrothermally in an autoclave at 100 °C for 16 h (pressure not monitored). The LDH so obtained was washed and dried under vacuum for further studies. Citric acid-coated Fe3O4 nanoparticles were synthesized using our previously published work.25 Towards this end, Fe3+ and Fe2+ (molar ratio 1.9
:
1) were precipitated at high pH using ammonia solution at 70 °C in an inert atmosphere. Citric acid was added to this solution and was incubated at 90–95 °C with mechanical stirring, in an inert atmosphere and under reflux. The nanoparticles obtained were thoroughly washed by magnetic decantation. The Fe3O4 nanoparticles were then suspended in MilliQ water and sonicated to prepare a ferrofluid for further studies. The hybrid nanomaterial was prepared by simple non-covalent electrostatic interactions mediated between the cationic LDH and anionic Fe3O4 nanoparticles as shown in Fig. 1. For this purpose, the as-prepared aqueous solutions of LDH and Fe3O4 nanoparticles were put in an ultrasonic water bath for 2 h (1
:
1 w/w) and maintained at room temperature. These magnetic nanohybrids (MNHs) were then collected over a strong permanent magnet and then dried under vacuum at room temperature.
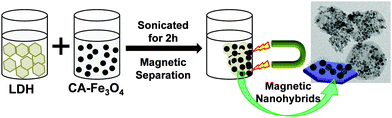 |
| Fig. 1 Schematic representation of synthesis of MNHs. | |
Calorimetric measurements
Time-dependent calorimetric measurements were performed on the aqueous suspensions of MNHs to evaluate their heating efficacy, and their SAR was calculated. The ACMF was generated using an RF generator that operated at a fixed frequency (425 kHz) in a solenoidal coil with appropriate insulation in order to minimize heat loss. 1 ml of a colloidal suspension of Fe3O4 and MNHs (1, 2 and 5 mg ml−1 Fe) was exposed to the ACMF with a field of 232, 271, 309, and 376 Oe, and the temperature rise was recorded. The SAR was calculated using the following equation:26 | 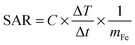 | (2) |
where C is the specific heat of the solvent (C = Cwater = 4.18 J g−1 °C), ΔT/Δt is the initial slope of the time versus temperature curve, and mFe is the mass fraction of iron in the samples. The parameters of the applied ACMF, time, and the concentration of Fe were optimized to reach and maintain the suspension temperature as 45 °C for further use in hyperthermia studies.
Drug loading and release
Doxorubicin hydrochloride (Dox), an established anticancer agent, was used as a model drug to evaluate the potential of the MNHs as delivery vehicles. In order to determine the drug-loading efficiency and the type of interactions of drug molecules with MNHs, the fluorescence spectra of pure Dox and Dox-loaded MNHs were studied. For this purpose, different amounts of MNHs (stock: 2 mg ml−1) were added to 1 ml of an aqueous Dox solution (10 μg ml−1) and mixed gently for 5 min. The fluorescence spectra of the supernatant (obtained after magnetic sedimentation of drug-loaded MNHs) were then recorded. The fluorescence spectrum of 1 ml of pure DOX (10 μg ml−1) was also recorded for comparative studies. The fluorescence intensities of the supernatants against that of the pure Dox solution were used to determine the loading efficiency of Dox molecules onto the MNHs. The loading efficiency (w/w%) was calculated using the following relation: |  | (3) |
where IDox is the fluorescence intensity of the pure Dox solution and IS is the fluorescence intensity of the supernatant. To determine the interactions between the MNHs and the Dox molecules, the fluorescence intensities were plotted against the total corresponding concentration of MNHs and analysed using the modified Stern–Volmer equation,27 | 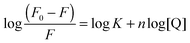 | (4) |
where F0 and F are the fluorescence intensities of Dox (fluorophore) in the absence and presence of MNHs (quencher), respectively, K is the binding constant, n is the binding affinity, and [Q] is the total concentration of MNHs.
The assessment of the drug release profile of the Dox-loaded MNHs was carried out under reservoir-sink conditions. For the release study, the amount of Dox-loaded MNHs was quantified according to their loading efficiency. The Dox-loaded MNHs were then magnetically decanted and re-suspended in 5 ml of sodium acetate buffer (pH 5), and then put into a dialysis bag. The dialysis was performed against 200 ml of phosphate buffered saline (PBS; pH 7.3) under continuous stirring at 37 °C, in order to mimic the cellular environment of lysosomes and cytoplasm. 1 ml aliquot of the sink (PBS) was withdrawn at fixed time intervals to be analysed for the amount of Dox released and was replaced with fresh PBS, simultaneously, to maintain the concentration gradient conditions. The aliquot was then subjected to the measurement of its fluorescence intensity, excited at 490 nm; the cumulative drug release was then calculated against the standard graph prepared under similar conditions (R2 = 0.998).
In-vitro studies
The toxicity of a functional nanomaterial toward a biological system is an important factor that limits the use of various potential carrier materials. To meet this end, a dose-dependent viability study was performed to evaluate the biocompatibility of these MNHs with murine fibroblast cells (L929) and human cervical cancer (HeLa) cells. For a typical experiment, the cells were seeded into 96-well plate at a density of 5 × 104 cells per well for 24 h. Then, 200 μl of serial dilutions of a colloidal suspension of MNHs (2.0, 1.0, 0.5, 0.25, 0.125, 0.0625, and 0.03125 mg ml−1) in an appropriate supplemented growth medium were added to the cells and incubated for an additional 24 h at 37 °C in a 5% CO2 environment. Thereafter, the cells were gently washed with PBS (pH 7.3) and then processed for the SRB assay to determine the viable cell population.28 Non-treated cells were used as control for the experiments. For a typical assay, the cells were fixed with 10% trichloroacetic acid solution by incubating at 4 °C for 1 h. The cells were then washed gently and stained by 0.4% SRB dissolved in 1% acetic acid and incubating in the dark. The cell-bound dye was then extracted with 200 μl of 10 mM Tris buffer solution (pH 10.5), and its optical density was measured using a multi-well plate reader at 560 nm. The viable cell population was calculated as a comparative against control using the following equation: |  | (5) |
The laser scanning confocal microscopic (LSCM) images were recorded to investigate the cellular internalization of the MNHs by HeLa cells. For the microscopy, the cells were seeded in a 30 mm sterile tissue culture Petri dish and incubated under physiological conditions (37 °C and 5% CO2 environment) for 24 h so that it could be grown as a monolayer. The cells were then incubated with the FITC-tagged MNHs that were suspended in the growth medium and incubated for a specific duration (3, 6, 12 and 24 h). Then, the cells were gently washed with PBS 3–4 times, fixed with 4% paraformaldehyde at room temperature for 10 min, and gently rinsed with PBS 3–4 times. In order to label the cell nuclei, a solution of propidium iodide (5 μg ml−1 in PBS) was added and incubated for 5 min, and then gently rinsed with PBS 3–4 times. They were then visualized and imaged using a confocal microscope.
Also, the amount of internalized MNHs was determined by the inductively coupled plasma-atomic emission spectroscopy (ICP-AES) analysis of the cell lysate. HeLa cells of 5 × 104 cell density were seeded in a sterile tissue culture Petri dish of 30 mm and incubated for 24 h under physiological conditions. The exhausted growth medium was then replaced by 1 ml of an aqueous suspension of MNHs (1 mg ml−1) that was mixed with supplemented growth medium; the cells were then incubated for 6 h under similar conditions. Subsequently, the cells were washed gently with PBS, trypsinized and suspended in 100 μl of sterile PBS. To this cell suspension, 500 μl of concentrated HCl was added and the cells were allowed to lyse for 10 min. The volume of the cell lysate was then made up to 10 ml with MilliQ water and was analysed for Fe2+, Mg2+ and Al3+ ions by atomic emission spectroscopy.
The therapeutic effect of Dox-loaded MNHs was investigated using HeLa cells. To this end, 5 × 104 cells were seeded in a 96-well plate and incubated at 37 °C in 5% CO2 environment for 24 h. Thereafter, varying amounts of Dox-loaded MNHs that were suspended in a supplemented growth medium were added to the wells after replacing the exhausted growth medium. The cells were further incubated under similar conditions for an additional 24 h. The SRB assay was performed to calculate the number of viable cells, as described earlier. The concentration of Dox-loaded MNHs that reduced the cell population by 50% was termed IC50 value; this value plays a significant role in the determination of the therapeutic dosage.
Treatment of cancer by magnetic hyperthermia
The heat-generating ability of the magnetic nanoparticles on exposure to an ACMF was used in the hyperthermic treatment of the cervical cancer cells. The fatal effect of magnetic heating by the MNHs was evaluated on these cells with and without the application of the ACMF. For a typical study, the HeLa cells were grown in a supplemented medium at 37 °C in a 5% CO2 environment. After the culture reached ∼90% confluency, the cells were detached from the culture flasks by treatment with a trypsin-EDTA solution, collected by centrifugation, and counted to a cell density of 1 × 106 by the trypan blue exclusion method.29 500 μl (2 mg ml−1) of sterile MNHs formulations were added to the cell suspensions and mixed gently after the volume was made up to 1 ml with the growth medium. The cell suspensions were then exposed to an ACMF of 376 Oe for initial 10 min to reach the hyperthermia temperature of 45 °C. The temperature of the suspension was maintained at 45 ± 0.5 °C; this exposure was continued for different treatment time intervals (10, 15, 20, 25 and 30 min). The selection of the applied ACMF was according to earlier optimization that was done by varying the applied field and the concentration of the MNHs. The cell suspension was then seeded in a 30 mm Petri dish with an additional 1 ml of supplemented growth medium and incubated for 6 h at 37 °C in a 5% CO2 environment. The cells were then washed gently with PBS, trypsinized, and counted for viable cell population by the trypan blue exclusion method. The viable cell population was represented as follows: |  | (6) |
The treatment time at which the cancer cell population is reduced to 50% is considered to be its median lethal dose time (LD50).
Results and discussion
Structural analyses
Fig. 2 shows the XRD patterns of Mg–Al LDH, Fe3O4 nanoparticles and MNHs. While the XRD pattern of LDH exhibited a typical rhombohedral (R
m) hydrotalcite crystal (JCPDS: 01-089-0460), the pattern of iron oxide was indexed as a cubic inverse spinel phase of Fe3O4 (JCPDS: 88-0866). All the characteristic diffraction peaks of LDH and Fe3O4 nanoparticles were observed in the MNHs sample. It is evident from the MNHs' pattern that the peaks of the Fe3O4 phase dominate, indicating the presence of Fe3O4 nanoparticles on the surface of LDH.
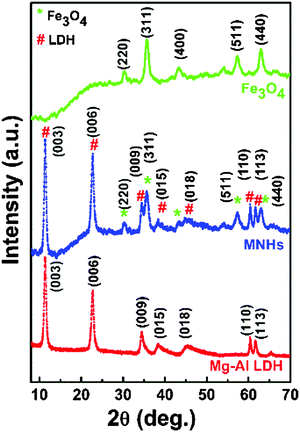 |
| Fig. 2 XRD patterns of Mg–Al LDH, Fe3O4 nanoparticles and MNHs. | |
Fig. 3 shows the electron micrographs of Fe3O4 nanoparticles, pure LDH and MNHs with their corresponding selected area electron diffraction (SAED) patterns (inset). Fig. 3a shows the Fe3O4 nanoparticles with essentially a spherical morphology and narrow size distribution. The particle size were in the range of 10–15 nm (σ ≤10%). Pure LDH (Fig. 3c) shows a hexagonal morphology with nearly circular edges that has a particle size of 80–100 nm. The SAED patterns that correspond to the diffraction planes of (003), (006) and (009) were indexed. Fig. 3e shows MNHs that have Fe3O4 nanoparticles that are well decorated and evenly distributed on the LDH surface. The SAED pattern of MNHs shows Fe3O4 as the primary phase and the (003) pattern of the LDH phase, which is in agreement with the XRD results.
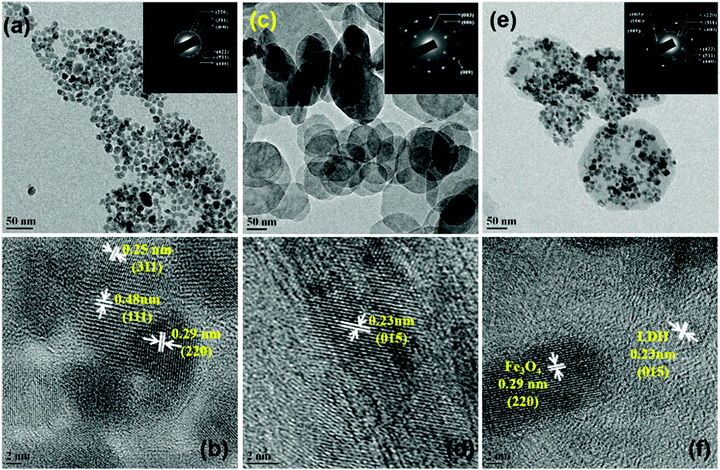 |
| Fig. 3 Transmission electron micrographs along with high resolution micrographs of (a and b) Fe3O4 nanoparticles, (c and d) LDH and (e and f) MNHs (insets depict the corresponding SAED patterns). | |
Surface analyses
Fig. 4 shows the infrared spectra of Fe3O4 nanoparticles, LDH and MNHs. The spectrum of Fe3O4 showed the successful conjugation of citric acid since it had the characteristic vibrations at 3135 cm−1 (C–H stretching), 1601 cm−1 (C
O stretching), 1385 cm−1 (COO− symmetric stretching), and 860–500 cm−1 (metal–oxygen vibrations). The evidence of carbonate intercalated in between the LDH phase is confirmed by the characteristic vibrational peaks that occur at 3553, 1646 cm−1 (–OH stretching), and 1370 cm−1 (–CO32− anions) and all metal–oxygen vibrations that are observed at 800–500 cm−1. The spectrum of MNHs indicates a mixture of LDH and Fe3O4 phases, with a meagre shift observed in the O–H and C–H stretching bands. This shift, along with the sharp peak at 1385 cm−1, indicates the attachment of Fe3O4 on the surface of LDH. A shoulder is seen at around 3000 cm−1 for LDH as well as for MNHs and is attributed to the hydrogen bonding between water molecules and carbonates in the interlayers of LDH sheets.30
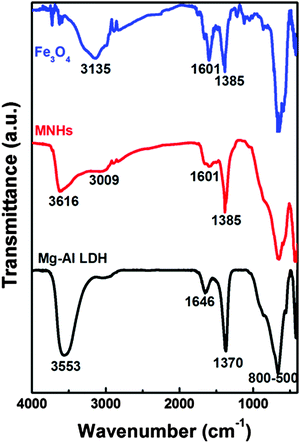 |
| Fig. 4 FTIR spectra of Mg–Al LDH, Fe3O4 nanoparticles and MNHs. | |
These nanomaterials were characterized for their hydrodynamic diameter by the dynamic light scattering (DLS) method (Fig. S2, ESI†). It was observed that the Fe3O4 nanoparticles had a size of 100 ± 4 nm while the LDH had a size of 210 ± 5 nm in aqueous environments with narrow size distributions. MNHs, on the other hand, had a broader size distribution with an average hydrodynamic size of 240 ± 9 nm. These numbers play an important role when these particles are considered for biological applications. The hydrodynamic diameter of MNHs is seen to be almost double of its absolute size obtained from electron micrographs. The interfacial water molecules interacting with the outer charged layer of MNHs contribute to this increase in size and also give us a better understanding of the fate of these particles in-vivo. The poly-dispersity index of Fe3O4 nanoparticles, LDH and MNHs is close to 0.2 (0.18, 0.18 and 0.23 respectively). This signifies that these particles are monodispersed and form stable aqueous suspension for biological applications.
The XPS spectra of citric acid-coated Fe3O4, LDH and MNHs were analysed for the C1s, O1s, Fe2p, Al2p, and Mg2p core levels. Fig. 5 shows the deconvoluted core levels for the MNHs, while the spectra of citric acid-coated Fe3O4 and LDH are thoroughly discussed in the ESI.† The C1s core levels were deconvoluted into three peaks that occur at 284.1, 285.3 and 288.1 eV, corresponding to C–C, carboxylate entities and carbonates, respectively. The peaks correspond to C–C and O–C
O vibrations that occur due to the citric acid groups of Fe3O4, while the carbonates are present in the interlayer spacing of the LDH layered structure. The O1s levels show two deconvoluted peaks at 529.4 and 532 eV, corresponding to carboxylate-linked oxygen/hydroxide/oxide and carbonate groups, respectively. The asymmetric features seen in the spectra indicate the presence of multiple oxygen bonds. The Fe2p levels reveal the presence of Fe2p3/2 and Fe2p1/2 oxidation states of iron oxide. The peak that occurs at 710.2 eV corresponds to the Fe–O bonds with oxygen of citric acid, while the peaks of Fe2p3/2 and Fe2p1/2 occur at 712.5 and 724 eV, respectively. A small-intensity, broad peak is also seen at 718 eV, corresponding to the Fe3+ oxidation state of iron. The Fe2p spectra of MNHs are consistent with the spectra of the citric acid-coated Fe3O4 nanoparticles, indicating that the formation of MNHs has not altered the structure of Fe3O4 in any way. The binding energy of the Al2p core level that arises at 74.2 eV and the symmetry in the spectra suggest the existence of Al3+ in the hydroxide form, which corroborates well with its spectra of the pure LDH sample. The core level of Mg2p is deconvoluted into two peaks at 54.6 and 56.4 eV to conclude that Mg2+ is linked by both oxide/hydroxide and carbonate entities. All the spectra show a shift in their binding energies, marking the successful conjugation of citric acid-coated Fe3O4 onto the surface of LDH layers.
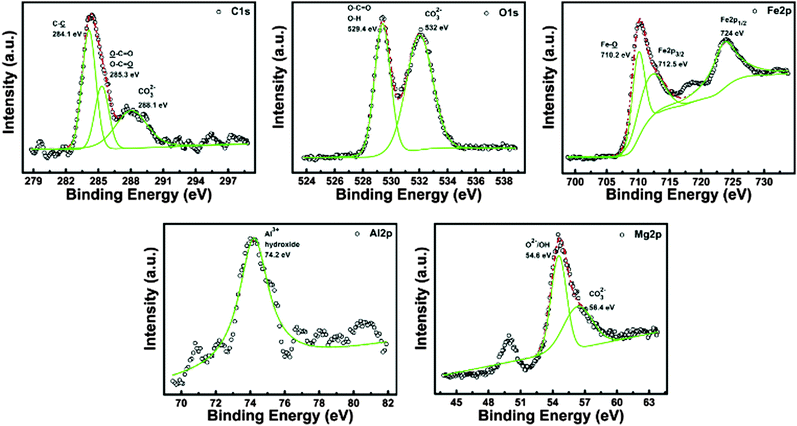 |
| Fig. 5 XPS spectra of MNHs. | |
The layers of the LDH sheets have the –OH groups in abundance, while the cations contribute toward their inherent positive charge. This charge-rich surface can readily interact with various nanomaterials, forming a variety of hybrid materials. This decoration of Fe3O4 nanoparticles on the surface of LDH is also evident by the zeta potential values of these nanomaterials. The negatively charged Fe3O4 and the positively charged LDH are expected to interact electrostatically to form the MNHs. Zeta potential values of LDH, Fe3O4 and MNHs are +37.9, −26.1 and −23.9 mV, respectively, which show a charge reversal for cationic LDH to anionic MNHs after decoration with Fe3O4. Most of the MNHs surface is covered with Fe3O4 with citric acid being exposed to the immediate microenvironment. This rendered a sufficiently negative zeta potential to MNHs, but slightly less in comparison to individual Fe3O4 nanoparticles.
Magnetic and calorimetric analyses
Fig. 6a shows the field-dependent magnetic measurements of Fe3O4 and MNHs at 300 K. Fe3O4 nanoparticles show superparamagnetic behaviour with a magnetization value of 69.1 emu g−1 at an applied field of 20 KOe, which lowered after its conjugation with LDH. The LDH nanoparticles constituted the non-magnetic components in the MNHs, thereby lowering the magnetization of MNHs to 44.4 emu g−1. The lowering in magnetisation of MNHs is about 36% for pure Fe3O4 nanoparticles. This is equivalent to the weight of LDH (∼40%) in MNHs indicated by the elemental analysis (Table S1, ESI†). The inset of the M–H curves shows negligible coercivity (∼10 Oe) which is a characteristic feature of superparamagnetism. And, ZFC-FC measurements were also performed to evaluate the shift in the blocking temperature (TB), if any, of the MNHs (Fig. S4, ESI†). The TB value of pure Fe3O4 nanoparticles and MNHs is found to be 260 and 230 K, respectively, and no change in the behaviour of the ZFC-FC curves was observed. This shift in TB, for MNHs, to a lower value is due to the non-magnetic contribution of LDH and decrease in the magnetic contribution of Fe3O4 nanoparticles. This in turn decreases the particle–particle interaction of Fe3O4 nanoparticles in nanohybrids.31 The magnetic measurements thus confirm that LDH is acting as a matrix to Fe3O4 nanoparticles in MNHs and are not contributing physically.
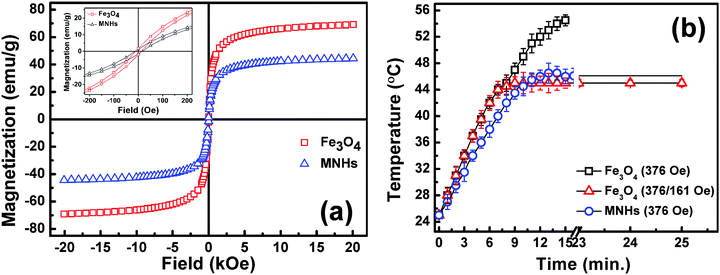 |
| Fig. 6 (a) M vs. H hysteresis loops of Fe3O4 and MNHs recorded at room temperature with low field inset and (b) calorimetric optimization and comparative performance of Fe3O4 nanoparticles with MNHs. | |
The generation of heat on exposure to an ACMF is a unique characteristic of magnetic nanoparticles owing to the Néel and Brownian relaxation losses. This property has been widely exploited by various researchers for treatment of cancer by hyperthermia.32–34 Time-dependent calorimetric measurements were performed on the aqueous suspensions of Fe3O4 and MNHs, and their SARs were found to be 98.4 and 73.88 W g−1 of Fe, respectively, at an applied field (H) of 376 Oe.
It was also observed that the time required to reach the hyperthermia temperature (45 °C) decreased with an increase in the applied field. This is in accordance with the fact that heat generation is proportional to the square of the applied ACMF (H).35,36 The data efficiently establish these MNHs as effective heating sources in hyperthermia treatment of cancer.
Fig. 6b shows the optimized time versus temperature graphs used for cellular magnetic hyperthermia studies. It was seen that at an applied field of 376 Oe, citric acid-coated Fe3O4 nanoparticles reach 45 °C within 6–7 min, but continue to generate heat, raising the temperature of the suspension up to 55 °C, which is detrimental to even normal cells. This challenge was addressed by applying a combination of ACMFs of varying strengths. A combination of field strengths of 376/161 Oe worked well to elevate the sample temperature to 45 °C and maintain it for a period of approximately 25 min. On the other hand, the MNHs required the magnetic field with a strength of 376 Oe for a similar performance, without any field reduction, and the MNHs acted as “cut-off switch” themselves as was required. It was observed that the MNHs reached the hyperthermia temperature within 10 min, and were capable of maintaining it for long treatment durations, making them a desirable candidate in clinical settings.
Drug loading and release
With LDH and Fe3O4 being established as functional materials that are biocompatible,37,38 the MNHs were evaluated for their capacity to carry a therapeutic payload to the desired tumour site. The fluorescence spectra of doxorubicin (Dox) were recorded to study the drug-loading efficiency of MNHs, which gave a clear picture of the interactions between Dox and MNHs. Fig. 7a shows the fluorescence spectra of an aqueous solution of pure Dox against the Dox-loaded MNHs. For a constant concentration of Dox in the solution, the concentration of MNHs was varied and a simultaneous quenching in the fluorescence was observed. Thus, it could be concluded that the increase in the concentration of MNHs is predominantly responsible for the significant quenching of the fluorescence. Since the fluorescence intensity of any fluorophore is largely dependent on its state, that is, free or bound, this decrease in the intensity is directly attributed to the binding interactions between MNHs and Dox and the subsequent reduction of the amount of free Dox in the solution. The positively charged –NH2 groups present in Dox are expected to bind electrostatically with the negatively charged carboxylate groups of citrate, which are decorated on the surface of Fe3O4.
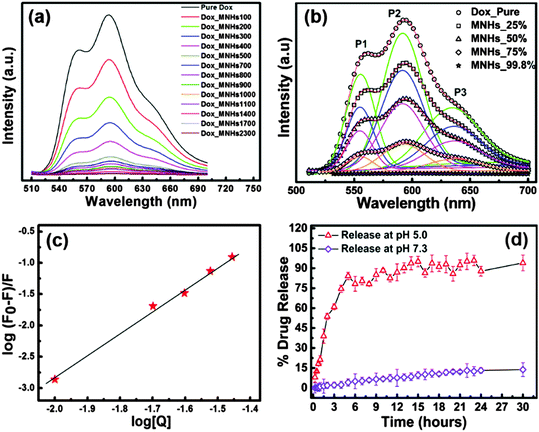 |
| Fig. 7 (a) Fluorescence spectrum of aqueous solution of pure Dox against the Dox-loaded MNHs, (b) deconvoluted Gaussian profiles at loading percentages of 25, 50, 75, and 99.8%, (c) modified Stern–Volmer plot for the drug conjugation with MNHs (R2 = 0.996) and (d) cumulative Dox release profile from MNHs in the pH environment of 5.0 and 7.3 over a period of 30 h. | |
Also, the presence of lone pairs of electrons on the ketone groups of Dox make them probable participating sites for hydrogen bonding with either the citrate ions or the positively charged LDH layers, if any. The loading efficiency of MNHs was calculated to be 99.8% (w/w). The binding interactions between the Dox molecules and the MNHs were further analysed and confirmed by multiple peak deconvolution by Gaussian curve fitting (Origin 8.0 software) (Fig. 7b). The fluorescence spectrum of Dox exhibits three emission maxima at 560 nm (P1), 590 nm (P2), and 630 nm (P3). The nature of the Dox–MNHs interaction is signified and revealed by a change in the peak maxima position, the shape of the spectrum, and the ratios of the area under each deconvoluted peak (A1/A2 and A3/A2).39 In comparison with the spectrum of a pure Dox solution, a gradual decrease is observed in the values of A1/A2 and A3/A2 for the Dox-loaded MNHs, which is a clear indication of the binding interactions between the two molecules (Table S2, ESI†).
Taking a step further in the understanding of drug–MNHs interactions, the modified Stern–Volmer plot was used to calculate the binding constant of the Dox molecules to the MNHs (Fig. 7c). The data were fitted by the method of least squares with a correlation factor (R2) of 0.996, using Origin 8.0 software. The y-intercept of plot represents the logarithmic value of the binding constant and was calculated to be 73.36 mg−1 ml−1 for MNHs. The slope of the plot is a representation of the binding affinity or the fraction of the fluorophore taking part in the interactions with the quencher molecule (here, MNHs), which was calculated to be 3.57 (linear data fit in Origin 8.0 software). This indicates that three of the possible binding sites in Dox are utilized for the conjugation with MNHs. The Gibbs free energy was calculated using the binding constant, resulting in a negative value (−11.07 kJ mol−1), which indicates spontaneous binding interactions between Dox and MNHs.
Fig. 7d shows the cumulative Dox release profile from the Dox-loaded MNHs under cell-mimicking conditions over a period of 30 h. The Dox release from MNHs shows a sustained release pattern for a period of initial 5–6 h (>50%), slowing down for the next 6 h and attaining a plateau thereafter (>95%). On the other hand, at near physiological pH (7.3) the MNHs release only ∼20% of the Dox even after 30 h. The results indicate that the MNHs could efficiently release Dox molecules in high proton environments as against neutral pH environments. This difference in the release percentage could be due to the decrease in the negative zeta potential of the MNHs in these acidic environments. This results in the protonation of the Dox molecules, which in turn leads to the weakening of the non-covalent binding interactions between Dox and MNHs. This weakening of the binding initiates the release of Dox into its immediate environment. This pH-stimulated drug release is desirable in cancer chemotherapy because the fluid surrounding the tumour cells is more acidic than the normal extracellular fluid. When the MNHs are delivered to the targeted cancer site, the release of Dox would be instigated, which would be further enhanced upon the particle internalization in the lysosomes of the cancer cells.
In-vitro studies
Biocompatibility and cell internalization studies.
The MNHs were evaluated for any toxic effects on cell proliferation or morphology of L929 and HeLa cells by incubating the cells in a medium that contained known concentrations of MNHs. The cells without any MNHs in the growth medium were considered as control. Fig. 8a shows the percentage of viable cells after treatment with the MNHs for a period of 24 h. Both L929 and HeLa cells are seen to be unaffected by the presence of MNHs in their growth medium for concentrations as high as 2 mg ml−1. The cationic nature of LDH might initiate the destabilization of the plasma membrane upon contact, which is counteracted by the presence of anionic Fe3O4 on its surface. Also, the tolerance and survival of HeLa cells toward these MNHs could be due to the anomaly in their structure, composition and function against normal cells, which aids in their survival.
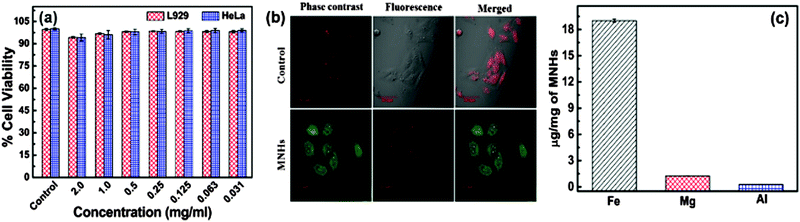 |
| Fig. 8 (a) Cell viability after treatment of 24 h with MNHs against L929 and HeLa cells, (b) LSCM images of cell internalization of MNHs after 6 h and (c) ICP-AES analysis of cell lysates for the quantification of Fe2+, Mg2+ and Al3+ ions. | |
Fig. 8b depicts the LSCM images captured in order to evaluate the cell internalization of the MNHs. The images show their successful internalization by HeLa cells after a period of 6 h. The uptake of MNHs by these cells was seen to initiate in 3 h, but the particles were scarcely internalized (data not shown), while at 6 h, the MNHs marked their presence not only in the cytoplasm but some of the MNHs could also be seen in the nuclei of the cells. The internalization of the MNHs does not reveal any undesired morphological changes in the cells marking its compatibility with the HeLa cells. Green fluorescence is present due to the FITC-tagged MNHs, while the nuclei are clearly stained by propidium iodide dye (red). Also, the successful cell internalization of MNHs was supported by the results of atomic emission spectroscopy (ICP-AES) (Fig. 8c). The amount of Fe2+, Mg2+ and Al3+ in the cells after 6 h, when compared to the control cells that were grown without MNHs in their growth medium, is found to be 19.0, 1.2, and 0.2 μg mg−1 of MNHs, respectively.
Therapeutic efficacy studies.
The Dox-loaded MNHs were evaluated for their therapeutic effect against HeLa cells over a period of 24 h (Fig. 9a). It was observed that the concentration of 0.94 mg ml−1 (R2 = 0.957) Dox-loaded MNHs competently reduced the viable cell population by 50% and hindered their proliferation. The IC50 value was calculated using the dose–response sigmoidal curve fitted using Origin 8.0 software. It could thus be deduced that the presence of MNHs affected the IC50 value of the Dox-loaded formulation and lowered the values when compared to the pure Dox molecules with a similar therapeutic efficacy. Also, from the cell internalization studies performed earlier (Fig. 8b) we can conclude that these MNHs are very much capable of carrying their therapeutic load inside the cells. As these MNHs are pH-sensitive (Fig. 7d), a higher amount of Dox is expected to be released inside the cells in lysosomes than the amount released outside in the extracellular fluid. Dox is then expected to diffuse from the lysosomal compartment to other parts of the cell thereby eliciting toxic effects in the cells.
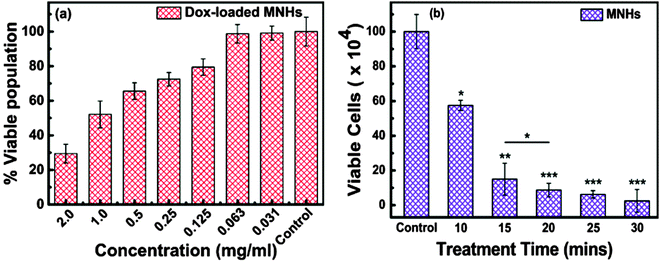 |
| Fig. 9 (a) Dose-dependent inhibition of cell proliferation of HeLa cells by Dox-loaded MNHs (IC50 evaluation) and (b) detrimental effects of ACMF on the viability of HeLa cells in the presence of MNHs (treatment times were found to be statistically significant against control cells with p < 0.05 (*), 0.01 (**) and 0.001 (***)). | |
Treatment of cancer by magnetic hyperthermia
The heat generated by the magnetic nanoparticles under the influence of an oscillating magnetic field, that is, an ACMF is widely utilized for the hyperthermia treatment of cancer under In-vitro as well as In-vivo conditions.40,41 The temperature for magnetic hyperthermia is desirable between 42 and 45 °C which is known to be fatal to cancer cells without being detrimental to neighbouring normal cells. Our developed MNHs were useful in elevating the temperature of the suspension from room temperature to 45 °C within 10 min of exposure to an ACMF of strength of 376 Oe (optimized earlier for different treatment times). Fig. 9b depicts the effect of the applied ACMF on the viable cell population of HeLa cells. It is seen that the live cell population decreases exponentially with the increase in the treatment time. Even a small treatment time of 10 min is sufficient to reduce the number of live cells by 60% and establish the efficiency of magnetic hyperthermia. Also, this fatal effect of heat on the viability of cells attains saturation at approximately 20 min and shows a minimal effect with further increase in the treatment time.
Conclusions
To conclude, the current work describes a simple facile approach for the fabrication of Fe3O4-decorated layered double hydroxide for use in thermo-chemotherapy of HeLa cells. These magnetic nanohybrids were thoroughly characterized and evaluated for their therapeutic efficacy. Doxorubicin was successfully loaded onto these nanohybrids, which showed an on-demand triggered release in a sustained fashion. The drug release was pH-augmented, which is a desired modality in cancer therapy due to the acidic tumour microenvironment. These carrier nanohybrids were seen to be biocompatible, with negligible toxicity. This magnetic nanohybrid platform was also assessed for its performance in the treatment of tumours by magnetic hyperthermia treatment through exposure to an AC magnetic field. The introduction of LDH in these magnetic nanohybrids acted as a “cut-off switch” for an otherwise uncontrolled heating of Fe3O4 nanoparticles. Overall, these newly fabricated nanohybrid platforms show promising In-vitro results, with enhanced therapeutic performance, and could be used to explore the combination of thermo-chemotherapy. The use of magnetic nanohybrids in thermo-chemotherapy, thus, promises to be a more effective approach in the treatment of cancer against the existing classical therapies.
Acknowledgements
EVPK and SN acknowledge DST and IITB-Monash Research Academy, respectively, for fellowships. The authors also acknowledge SAIF, IIT Bombay for material characterization and the Department of Physics, IIT Bombay for XPS measurements. EVPK acknowledges the Department of Science and Technology for financial assistance for the current work.
References
- G. Delaney, S. Jacob, C. Featherstone and M. Barton, Cancer, 2005, 104, 1129–1137 CrossRef PubMed.
- M. A. Dawson and T. Kouzarides, Cell, 2012, 150, 12–27 CrossRef CAS PubMed.
- T. Vial and J. Descotes, Toxicology, 2003, 185, 229–240 CrossRef CAS PubMed.
- K. Cho, X. Wang, S. Nie, Z. Chen and D. M. Shin, Clin. Cancer Res., 2008, 14, 1310–1316 CrossRef CAS PubMed.
- S. Laurent, D. Forge, M. Port, A. Roch, C. Robic, L. Vander Elst and R. N. Muller, Chem. Rev., 2008, 108, 2064–2110 CrossRef CAS PubMed.
- E. Katz and I. Willner, Angew. Chem., Int. Ed., 2004, 43, 6042–6108 CrossRef CAS PubMed.
- S. Chandra, K. C. Barick and D. Bahadur, Adv. Drug Delivery Rev., 2011, 63, 1267–1281 CrossRef CAS PubMed.
- R. E. Rosensweig, J. Magn. Magn. Mater., 2002, 252, 370–374 CrossRef CAS.
- Q. A. Pankhurst, J. Connolly, S. K. Jones and J. Dobson, J. Phys. D: Appl. Phys., 2003, 36, R167 CrossRef CAS.
- S. Laurent, S. Dutz, U. O. Häfeli and M. Mahmoudi, Adv. Colloid Interface Sci., 2011, 166, 8–23 CrossRef CAS PubMed.
- D. Ling and T. Hyeon, Small, 2013, 9, 1449 CrossRef CAS.
- R. Love, R. Z. Soriano and R. J. Walsh, Cancer Res., 1970, 30, 1525–1533 CAS.
- B. Zümreoglu-Karan and A. Ay, Chem. Pap., 2012, 66, 1–10 CrossRef.
- A. I. Khan, L. Lei, A. J. Norquist and D. O'Hare, Chem. Commun., 2001, 2342–2343, 10.1039/B106465G.
- H. S. Panda, R. Srivastava and D. Bahadur, J. Phys. Chem. B, 2009, 113, 15090–15100 CrossRef CAS PubMed.
- V. Rives, M. del Arco and C. Martín, Appl. Clay Sci., 2014, 88–89, 239–269 CrossRef CAS.
- Y. Kuthati, R. K. Kankala and C.-H. Lee, Appl. Clay Sci., 2015, 112–113, 100–116 CrossRef CAS.
- Q. Wang and D. O'Hare, Chem. Rev., 2012, 112, 4124–4155 CrossRef CAS PubMed.
- G. Abellán, C. Martí-Gastaldo, A. Ribera and E. Coronado, Acc. Chem. Res., 2015, 48, 1601–1611 CrossRef PubMed.
- S.-J. Choi, J.-M. Oh and J.-H. Choy, J. Inorg. Biochem., 2009, 103, 463–471 CrossRef CAS PubMed.
- B. Saifullah, P. Arulselvan, M. E. El Zowalaty, S. Fakurazi, T. J. Webster, B. M. Geilich and M. Z. Hussein, Int. J. Nanomed., 2014, 9, 4749–4762 Search PubMed.
- L. Li, W. Gu, J. Chen, W. Chen and Z. P. Xu, Biomaterials, 2014, 35, 3331–3339 CrossRef CAS PubMed.
- M.-Q. Zhao, Q. Zhang, J.-Q. Huang and F. Wei, Adv. Funct. Mater., 2012, 22, 675–694 CrossRef CAS.
- Z. P. Xu, G. S. Stevenson, C.-Q. Lu, G. Q. Lu, P. F. Bartlett and P. P. Gray, J. Am. Chem. Soc., 2006, 128, 36–37 CrossRef CAS PubMed.
- S. Nigam, K. C. Barick and D. Bahadur, J. Magn. Magn. Mater., 2011, 323, 237–243 CrossRef CAS.
- M. Babincová, D. Leszczynska, P. Sourivong, P. Čičmanec and P. Babinec, J. Magn. Magn. Mater., 2001, 225, 109–112 CrossRef.
- B. Ahmad, S. Parveen and R. H. Khan, Biomacromolecules, 2006, 7, 1350–1356 CrossRef CAS PubMed.
- V. Vichai and K. Kirtikara, Nat. Protoc., 2006, 1, 1112–1116 CrossRef CAS PubMed.
-
W. Strober, Current Protocols in Immunology, John Wiley & Sons, Inc., 2001 Search PubMed.
- T. Hiblno, Y. Yamashita, K. Kosuge and A. Tsunashima, Clays Clay Miner., 1995, 43, 427–432 Search PubMed.
- K. Rumpf, P. Granitzer, P. M. Morales, P. Poelt and M. Reissner, Nanoscale Res. Lett., 2012, 7, 1–4 CrossRef PubMed.
- K. Hayashi, K. Ono, H. Suzuki, M. Sawada, M. Moriya, W. Sakamoto and T. Yogo, ACS Appl. Mater. Interfaces, 2010, 2, 1903–1911 CAS.
- M. N. Luwang, S. Chandra, D. Bahadur and S. K. Srivastava, J. Mater. Chem., 2012, 22, 3395–3403 RSC.
- M. Johannsen, B. Thiesen, P. Wust and A. Jordan, Int. J. Hyperthermia, 2010, 26, 790–795 CrossRef PubMed.
- Y. Piñeiro-Redondo, M. Bañobre-López, I. Pardiñas-Blanco, G. Goya, M. A. López-Quintela and J. Rivas, Nanoscale Res. Lett., 2011, 6, 383 CrossRef PubMed.
- P. de la Presa, Y. Luengo, M. Multigner, R. Costo, M. P. Morales, G. Rivero and A. Hernando, J. Phys. Chem. C, 2012, 116, 25602–25610 CAS.
- S. J. Choi, J. M. Oh and J. H. Choy, J. Nanosci. Nanotechnol., 2010, 10, 2913–2916 CrossRef CAS PubMed.
- Y. Liu, Z. Chen and J. Wang, J. Nanopart. Res., 2011, 13, 199–212 CrossRef CAS.
- K. C. Barick, S. Nigam and D. Bahadur, J. Mater. Chem., 2010, 20, 6446–6452 RSC.
- T.-J. Li, C.-C. Huang, P.-W. Ruan, K.-Y. Chuang, K.-J. Huang, D.-B. Shieh and C.-S. Yeh, Biomaterials, 2013, 34, 7873–7883 CrossRef CAS PubMed.
- T. Sadhukha, T. S. Wiedmann and J. Panyam, Biomaterials, 2013, 34, 5163–5171 CrossRef CAS PubMed.
Footnotes |
† Electronic supplementary information (ESI) available. See DOI: 10.1039/c5nj01701g |
‡ Authors have equal contribution towards this work. |
|
This journal is © The Royal Society of Chemistry and the Centre National de la Recherche Scientifique 2016 |
Click here to see how this site uses Cookies. View our privacy policy here.