DOI:
10.1039/C5NJ02349A
(Paper)
New J. Chem., 2016,
40, 372-376
Convenient preparation of Pd/RGO catalyst for the efficient hydrodechlorination of various chlorophenols†
Received
(in Nottingham, UK)
2nd September 2015
, Accepted 24th October 2015
First published on 27th October 2015
Abstract
Uniformly dispersed Pd nanoparticles supported on reduced graphene oxide (RGO) have been conveniently synthesized by a facile one-pot approach through the co-reduction of sodium tetrachloropalladate(II) and graphene oxide (GO) using NaBH4 as the reducing agent. The catalytic activity of Pd/RGO was investigated for aqueous hydrodechlorination (HDC) of chlorophenols at room temperature using hydrogen balloon pressure without any additives. Results indicated that Pd/RGO exhibited an excellent activity with a reaction rate of 280.0 min−1 gpd−1 for the hydrodechlorination (HDC) of 4-CP. Furthermore, the Pd nanoparticles supported on RGO can be easily recovered and reused for four times without any obvious leaching and loss of activity.
1. Introduction
Chlorophenols (CPs) have been widely used as pesticides, fungicides, herbicides, dyes, solvents, disinfectants and wood preservatives in industry and agriculture.1,2 However, the CPs residues can have adverse effect on the environment because CPs are barely biodegraded or self-cleaned in the environment. Nowadays, various techniques have been adopted for the disposal of residual CPs, among which catalytic hydrodechlorination (HDC) has been demonstrated as a cost-effective and environment-friendly technology.3 The HDC of CPs using various heterogeneous catalysts containing noble metals (e.g., Pd,1,3–6 Rh,2,7–11 and Pt2,7) has attracted tremendous interest due to its high reactivity.
Catalyst support plays a critical role in enhancing the activity of noble metals due to the improved dispersion of metal nanoparticles (NPs). Pd NPs deposited on conventional carriers, i.e., active carbon,12 Al2O32,8 and pillared clays,7,9 have been tested as catalysts for CPs degradation. Recently, graphene has been identified as an excellent stabilizer11 due to its excellent tolerance to HCl and high ability to improve the dispersion of metal NPs. Although Pd/RGO has good reactivity towards the 4-CP HDC, the Pd/RGO preparation has been tedious and the reduction conditions of GO have been rigorous to some extent. In the current study, a facile one-pot synthetic method was developed to prepare Pd/RGO NPs via the co-reduction of sodium tetrachloropalladate(II) and GO using sodium borohydride. The as-prepared Pd/RGO catalyst was found to exhibit high activity and stability in the HDC of 4-CP under moderate conditions.
2. Experimental section
2.1 Reagents and materials
Na2PdCl4·6H2O was purchased from the Kunming Institute of Precious Metals, China. Graphite powder and sodium borohydride (NaBH4) were obtained from Aladdin Industrial Inc., China. Other solvents were of analytical grade and used as received.
Graphene oxide (GO) was prepared according to the improved Hummers method13.
Typically, graphite powder (3.0 g) and KMnO4 (18.0 g) were transferred into a 500 mL round-bottom flask. Then, a mixture of concentrated H2SO4/H3PO4 (360
:
40 mL) was slowly added dropwise to the flask with vigorous stirring. The mixture was heated to 50 °C, stirred for another 24 h and then 400 mL of ice containing 3 mL of 30% H2O2 was poured into the flask. The resulting suspension was centrifuged and washed several times with deionized water, HCl (36 wt%), and absolute ethanol. The desired product was obtained as a solid and was dried under vacuum at room temperature for 12 h.
Pd/RGO catalyst was synthesized using a one-pot co-reduction method described as follows.
GO (150 mg) was dispersed in 50 mL of deionized water under ultrasonication to obtain a uniform suspension, in which 2.88 mL of Na2PdCl4 with a Pd concentration of 1.61 mg mL−1 was slowly added with continuous stirring. Subsequently, 10 mL of an aqueous solution of NaBH4 (150 mg) was added dropwise into the mixture at 0 °C. The resulting black solid was collected by centrifugation and washed several times with water and absolute ethanol. Finally, the black solid was dried under vacuum at 60 °C for 12 h, yielding the desired Pd/RGO catalyst.
2.2 Activity tests
The catalytic activity of Pd/RGO towards the degradation of CPs was evaluated at 25 °C under hydrogen balloon pressure. Typically, 5.0 mg of Pd/RGO was added into a 25 mL two-necked round-bottom flask equipped with a hydrogen balloon. Then, 5.0 mL of 4-CP aqueous solution with a 4-CP concentration of 2.5 g L−1 was transferred into the flask with continuous stirring. The catalyst concentration was kept at 1.0 g L−1. The reaction temperature was controlled using a water bath. The flask was purged several times with pure hydrogen to remove the air. The reaction time was set at zero, when the temperature of reactant solution was stable. During the course of the reaction, samples were withdrawn from the flask periodically using a syringe and analyzed by GC (Agilent 7890A) equipped with a FID detector and PEG-20M capillary column (30 m × 0.25 mm, 0.25 μm film). Nitrogen acted as the carrier gas.
3. Results and discussion
3.1 Characterization of the Pd/RGO catalyst
The TEM image (Fig. 1(a)) of Pd/RGO with a Pd loading of 3.0 wt% demonstrates that Pd NPs are uniformly dispersed on the surface of RGO sheets, which is probably assigned to the interaction between the Pd ions and oxygen-containing groups of GO that inhibit the aggregation of Pd NPs during the reduction process. However, as shown in Fig. 1(b), Pd/RGO NPs begin to aggregate after the fifth run. The strong diffraction peak at 2θ = 26.4° of graphite shown in the inset of Fig. 1(c) was found to disappear and a new peak at 2θ = 10.0° corresponding to the plane of GO appeared. Thus, the successful oxidation of graphite to GO was clearly confirmed. In addition, the disappearance of the characteristic peaks of GO suggests the efficient reduction of GO. The two new peaks at the position of 25.4° and 43.1° are attributed to the (002) and (100) reflections of RGO, respectively. The new peaks of Pd/RGO at 2θ = 39.1°, 45.4°, and 66.1°, which correspond to the (111), (200), and (220) planes of Pd (JCPDS card no. 87-0637), demonstrate that the Pd NPs are indeed loaded on the RGO surface through the one-pot co-reduction procedure.
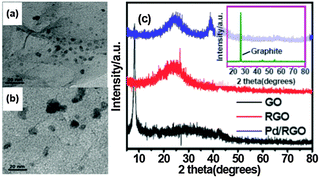 |
| Fig. 1 TEM images of Pd/RGO (a) and Pd/RGO after five runs (b) as well as XRD patterns of GO, RGO and Pd/RGO (c). The inset of (c) displays the XRD pattern of graphite. | |
To determine the surface compositions and the electronic states of each element on the surface of Pd/RGO, the XPS elemental survey scans of Pd/RGO were first conducted (Fig. 2). The results obtained show the existence of Pd, oxygen, and carbon on the RGO surface. The C1s spectrum could be deconvoluted into four peaks, which were attributed to C–C, C–OH, C–O–C and HO–C
O. The declined peak intensities of the oxygen-containing groups on the RGO surface suggest the successful reduction of GO (Fig. 2(b) and (c)). The binding energy of the Pd3d5/2 level in Pd/RGO is 335.8 eV, which is higher than that of the standard zero-valent state of Pd (335.0 eV). The increase in binding energy of Pd(0) results from the electron transformation from palladium to RGO due to the strong interaction between Pd and RGO.14 The peak at 337.9 eV is assigned to the oxidation state of Pd species,15 and to some extent the presence of the oxidation state of Pd facilitates the HDC of 4-CP, as reported in a study by Dr Seoane.16
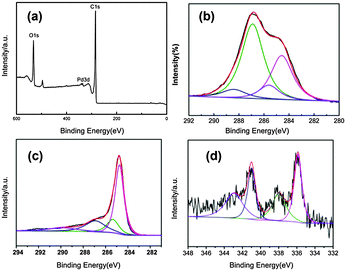 |
| Fig. 2 XPS scans of Pd/RGO (a); XPS spectra of C1s peaks of GO (b) and RGO (c) and Pd 3d peaks of Pd/RGO (d). | |
In fact, the facts mentioned above were also verified by the changed FT-IR adsorptions of the surface oxygen-containing functional groups on GO and RGO-supported Pd NPs (Fig. 3). Namely, for GO, the broad characteristic band at 3420 cm−1 is attributed to the O–H stretching vibration arising from hydroxyl groups. The absorption band associated with C
O stretching is at 1730 cm−1, that for C–O stretching is at 1040 cm−1 and the C
C peak from unoxidized sp2 C–C bonds are detected at 1610 cm−1. The absorption peaks at 1230 cm−1 and 1040 cm−1 are attributed to the C
O vibrations and C–O groups situated at the edges of GO, respectively. This testifies the fact that the oxygen-containing groups on the GO surface provide reactive anchoring sites for Pd ions and inhibit the aggregation of Pd NPs during the reduction process. GO is successfully reduced in the aqueous phase using NaBH4, the evidence for which is provided by the decreased intensity of the peaks corresponding to the oxygen-containing groups. Accordingly, based on the above-mentioned characterization, we believe that the desired Pd/RGO catalyst was obtained using the convenient co-reduction process.
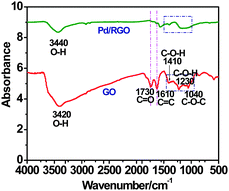 |
| Fig. 3 The distinguishable FT-IR adsorptions of GO and RGO-supported Pd NPs. | |
3.2 Effect of various solvents on the HDC of 4-chlorophenol
In this case, the effect of various solvents, including water, methanol, ethanol, cyclohexane and 1,4-dioxane, on the catalytic performance of Pd/RGO was first evaluated for the HDC of 4-CP (Fig. 4). It can be seen that Pd/RGO displayed distinguishable reactivity in the various solvents. In particular, 4-CP could be completely converted to phenol in 90 min when water acted as a green solvent. Moreover, the reactivities of Pd/RGO in protic solvents and aprotic solvents were obviously decreased. The initial HDC rates of 4-CP over Pd/RGO in the different solvents exhibited a significant dependence on the reaction media and decreased in the following order: water > methanol > ethanol > cyclohexane > 1,4-dioxane.
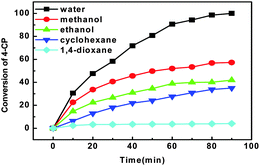 |
| Fig. 4 Effect of different solvents on the conversion of 4-CP. | |
With respect to the product distributions, the main product was phenol and only a trace amount of cyclohexanone (<0.1%) was detected. This fact was probably attributed to the low concentration of catalyst (1.0 g L−1) and high initial concentration of 4-CP (2.5 g L−1) as well as the low reaction temperature (25 °C).17 In addition, the low reaction temperature also contributed to the low selectivity to cyclohexanone,17 which was confirmed by observing an increase in cyclohexanone selectivity to 0.9% when the reaction was conducted at an elevated temperature of 40 °C (Fig. S1, ESI†). Furthermore, the product distributions did not obviously vary regardless of the solvent used. A solvent with a high dielectric constant and low molar volume is beneficial to the HDC reaction. On the one hand, a solvent with a higher dielectric constant provides stronger ionic forces to stabilize the electropositive arenium intermediate. On the other hand, a solvent with a low molar volume means more solvent molecules are available to interact with the charged reaction intermediate, which obviously accelerates the HDC reaction of 4-CP. Thus, the highest catalytic activity of Pd/RGO for the HDC of 4-CP in water was ascribed to the properties of water. In comparison with the other four organic media, the highest activity of Pd/RGO for the HDC of 4-CP in water was due to the well-organized structure of water originating from the formation of H-bonding between the dissolving ions and water with the highest/lowest values of dielectric constant and molar volume.18
3.3 Kinetics and recycling test of the catalyst
As illustrated in Fig. 5, the kinetic study showed that the conversion of 4-CP increased with an elevation of reaction temperature in the range from 298 to 313 K. Moreover, there is no noticeable change towards the product distribution regardless of the temperature variation. Rate constants (kobs) are determined from the slope of the linear part of each plot, as shown in Fig. 5(a). The activation energy (Ea) is calculated using the Arrhenius equation (eqn (1)). Consequently, the activation energy is estimated to be 61.5 kJ mol−1 based on the slope of the ln
k−(1/T) plot shown in Fig. 5(b). The kinetic reaction rate of 4-CP HDC catalyzed by Pd/RGO was compared with the results reported in the literature. The Pd/Al2O3 catalyst reported by Díaz et al.2 showed a kinetic reaction rate of 3.33 min−1 g−1 and Pd/pillared clays reported by Molina et al.7 exhibited a reaction rate of 7.6 min−1 g−1. An increase in the reaction rate to 13.2 min−1 g−1 was detected by Liu et al.17 using a Pd/dendritic mesoporous silica nanospheres. Notably, in our case the highest reaction rate of 280.0 min−1 g−1 was observed at the same reaction temperature when the HDC of 4-CP was catalyzed by Pd/RGO, which was probably ascribed to the strong interaction between Pd and RGO as well as the presence of electron-deficient Pd on the RGO surface. | kobs = A e−Ea/RT | (1) |
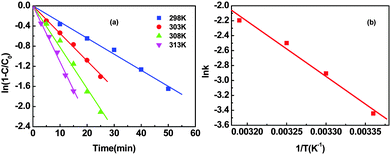 |
| Fig. 5 Effect of temperature on the reactivity of Pd/RGO towards the HDC of 4-CP (a). The dependence of ln k upon 1/T based on the rearranged Arrhenius equation (b). | |
The stability and recyclability of Pd/RGO for the HDC of 4-CP was investigated with successive runs under the operating conditions. After each run, the solid catalyst was separated from the reactor by centrifugation and the supernatant liquid was removed. The black solid was thoroughly washed three times with ethanol, dried in vacuum and reused for the next run. As shown in Fig. 6, the catalyst is quite stable and no loss of activity for the HDC of 4-CP was detected after the catalyst was reused four times. However, the activity of Pd/RGO began to decrease after the fifth run. A Pd leaching test was firstly conducted using ICP and the results indicate that the Pd leaching is negligible (0.4 ppm). In addition, an XRD investigation shows that the crystalline structure of the recovered catalyst is mostly retained after five catalytic cycles (Fig. S2, ESI†). The surface properties of the recycled catalyst analyzed by XPS (Fig. S3, ESI†) shows that the binding energy of Pd(0) in the recovered catalyst was shifted to 334.5 eV, which was probably due to the inhibition of electron transfer from palladium to RGO, resulting in a decrease in the interaction between Pd and RGO. The TEM image of the recycled catalyst indicates the aggregation of Pd NPs during the recycling process (Fig. 1(b)). Furthermore, the elemental mapping (Fig. S4, ESI†) of metallic Pd on the Pd/RGO catalyst provides available and visual evidence for the aggregation and loss of Pd after five runs even if the corresponding SEM images do not show observable changes. Therefore, a decrease in the interactions between Pd and RGO and the increase in Pd particle size were responsible for the loss of catalytic activity in the fifth run. Further studies should be carried out to improve the reusability of the Pd/RGO catalyst for practical applications.
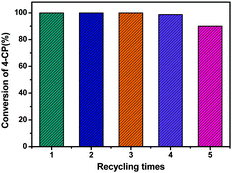 |
| Fig. 6 Recyclability survey of Pd/RGO for the HDC of 4-CP. | |
3.4 Reactivity of Pd/RGO for the HDC of various CPs
To evaluate the activity of the as-prepared Pd catalyst for the HDC of different substrates, the catalytic performance of Pd/RGO was screened for the HDC of five other types of chlorophenols (Table 1). The observations indicate that the reaction time for the complete conversion of monochlorophenols increases in the order of 3-CP < 2-CP < 4-CP, which probably results from the distinguishable electronic and steric hindrance of these monochlorine-substituted substrates. In addition, dichlorophenols (2,4-DCP and 2,6-DCP) and trichlorophenol (2,4,6-TCP) can be completely subjected to HDC to produce phenol as the reaction time was prolonged. In brief, the HDC rates of the CPs decrease in the following order: monochlorophenols > dichlorophenols > trichlorophenol, which is consistent with a previous study.19 The increased reaction time with an increase in the substitution of the substrate was probably assigned to the poisoned active sites of the Pd/RGO catalyst because of the higher content of HCl generated during the reaction process.
Table 1 HDC of different CPs catalyzed by Pd/RGO
Substrate |
Product |
Conv. (%) |
Time (min) |
Reaction conditions: temperature: 25 °C, pressure: hydrogen balloon pressure, metal concentration: 1.0 g L−1, and 4-CP concentration: 2.5 g L−1 (total volume: 5.0 mL). |
2-CP |
Phenol |
100 |
70 |
3-CP |
Phenol |
100 |
60 |
2,4-DCP |
Phenol |
100 |
120 |
2,6-DCP |
Phenol |
100 |
150 |
2,4,6-TCP |
Phenol |
100 |
200 |
4. Conclusions
In summary, Pd/RGO synthesized by a facile one-pot method exhibited excellent reactivity for the HDC of various chlorophenols. Moreover, the as-prepared Pd/RGO can be reused at least four times without any significant deactivation. In particular, in environmentally friendly solvent, namely, water, the complete conversion of 4-chlorophenol was achieved using the Pd/RGO catalyst in 90 min under moderate conditions. This advanced composite material provides a type of effective catalyst with great promise for the catalytic HDC of CPs in practical applications. Moreover, the novel preparation strategy involving a one-pot co-reduction reaction will attract the attention of researchers.
Acknowledgements
We gratefully thank the financial support given by the Applied Basic Research Program of Science and Technology Department of Sichuan Province (2014JY0107) and the National Natural Science Foundation of China (21207109).
Notes and references
- H. Rong, S. Cai, Z. Niu and Y. Li, ACS Catal., 2013, 3, 1560–1563 CrossRef CAS.
- E. Díaz, J. A. Casas, Á. F. Mohedano, L. Calvo, M. A. Gilarranz and J. J. Rodríguez, Ind. Eng. Chem. Res., 2008, 47, 3840–3846 CrossRef.
- C. Xia, Y. Liu, S. Zhou, C. Yang, S. Liu, J. Xu, J. Yu, J. Chen and X. Liang, J. Hazard. Mater., 2009, 169, 1029–1033 CrossRef CAS PubMed.
- C. B. Molina, L. Calvo, M. A. Gilarranz, J. A. Casas and J. J. Rodríguez, J. Hazard. Mater., 2009, 172, 214–223 CrossRef CAS PubMed.
- C. Xia, Y. Liu, S. Zhou, C. Yang, S. Liu, S. Guo, Q. Liu, J. Yu and J. Chen, Catal. Commun., 2009, 10, 1443–1445 CrossRef CAS.
- H. Hildebrand, K. Mackenzie and F.-D. Kopinke, Environ. Sci. Technol., 2009, 43, 3254–3259 CrossRef CAS PubMed.
- C. B. Molina, A. H. Pizarro, J. A. Casas and J. J. Rodríguez, Appl. Catal., B, 2014, 148–149, 330–338 CrossRef CAS.
- M. Munoz, Z. M. de Pedro, J. A. Casas and J. J. Rodríguez, Appl. Catal., A, 2014, 488, 78–85 CrossRef CAS.
- A. H. Pizarro, V. M. Monsalvo, C. B. Molina, A. F. Mohedano and J. J. Rodriguez, Chem. Eng. J., 2015, 273, 363–370 CrossRef CAS.
- J. A. Baeza, L. Calvo, M. A. Gilarranz and J. J. Rodriguez, Chem. Eng. J., 2014, 240, 271–280 CrossRef CAS.
- G. Y. Fan, Y. L. Ren, W. D. Jiang, C. Y. Wang, B. Xu and F. A. Liu, Catal. Commun., 2014, 52, 22–25 CrossRef CAS.
- L. Calvo, M. A. Gilarranz, J. A. Casas, A. F. Mohedano and J. J. Rodríguez, Appl. Catal., B, 2006, 67, 68–76 CrossRef CAS.
- D. C. Marcano, D. V. Kosynkin, J. M. Berlin, A. Sinitskii, Z. Sun, A. Slesarev, L. B. Alemany, W. Lu and J. M. Tour, ACS Nano, 2010, 4, 4806–4814 CrossRef CAS PubMed.
- W. J. Shen, M. Okumura, Y. Matsumura and M. Haruta, Appl. Catal., A, 2001, 213, 225–232 CrossRef CAS.
- R. Gopinath, N. Seshu Babu, J. Vinod Kumar, N. Lingaiah and P. S. Sai Prasad, Catal. Lett., 2008, 120, 312–319 CrossRef CAS.
- L. M. Gómez-Sainero, X. L. Seoane, J. L. G. Fierro and A. Arcoya, J. Catal., 2002, 209, 279–288 CrossRef.
- Y. S. Liu, Z. P. Dong, X. L. Li, X. D. Le, W. Zhang and J. T. Ma, RSC Adv., 2015, 5, 20716–20723 RSC.
- S. Gómez-Quero, F. Cárdenas-Lizana and M. A. Keane, AIChE J., 2010, 56, 756–767 Search PubMed.
- Y. L. Ren, G. Y. Fan, W. D. Jiang, B. Xu and F. A. Liu, RSC Adv., 2014, 4, 25440–25446 RSC.
Footnote |
† Electronic supplementary information (ESI) available. See DOI: 10.1039/c5nj02349a |
|
This journal is © The Royal Society of Chemistry and the Centre National de la Recherche Scientifique 2016 |
Click here to see how this site uses Cookies. View our privacy policy here.