DOI:
10.1039/C5NR05286F
(Paper)
Nanoscale, 2016,
8, 276-282
Thiazole derivative-modified upconversion nanoparticles for Hg2+ detection in living cells†
Received
5th August 2015
, Accepted 11th November 2015
First published on 12th November 2015
Abstract
Mercury ion (Hg2+) is an extremely toxic ion, which will accumulate in human bodies and cause severe nervous system damage. Therefore, the sensitive and efficient monitoring of Hg2+ in human bodies is of great importance. Upconversion nanoparticle (UCNPs) based nano probes exhibit no autofluorescence, deep penetration depth and chemical stability in biological samples, as well as a large anti-stokes shift. In this study, we have developed thiazole-derivative-functionalized UCNPs, and employed an upconversion emission intensity ratio of 540 nm to 803 nm (I540/I803) as a ratiometric signal to detect Hg2+ in living cells showing excellent photo stability and high selectivity. Our nano probe was characterized using transmission electron microscopy (TEM) and powder X-ray diffraction (PXRD). The low cytotoxicity of our probe was confirmed by an MTT assay and the UCL test in HeLa cells was carried out by confocal microscopy. Our results demonstrated that organic-dye-functionalized UCNPs should be a good strategy for detecting toxic metal ions when studying cellular biosystems.
Introduction
Detection of the structural and functional properties in living systems has been a perennial key challenge.1–8 For instance, diagnosis and treatment of cancer necessitates highly contrasted real-time bioimaging.9–11 Conventional luminescent materials such as organic dyes and quantum dots12 (QDs) have been explored in the past decades. However, these biolabels have some limitations. Usually the emission lifetimes of organic dyes are short (less than 100 ns),13 which are difficult to differentiate from other short-lived autofluorescence from biological samples. QDs have high toxicity and a short circulation half time,14 which will limit their bioapplication. Therefore, lanthanide doped upconversion nanoparticles (UCNPs) have been suggested as a promising biolabel.
Usually the energy of fluorescent photons is higher than that of excitation ones. However, lanthanide-doped UCNPs can convert near-infrared (NIR) excitation into visible emission of different colors,15–17 with a large anti-stokes shift in the hundreds of nanometers.18–20 UCNPs have many benefits such as chemical stability,21,22 non-autofluorescence from biological samples,21–25 remarkable light penetration depth,23,26–28 long lifetime (millisecond time scale)19,29 and less damage to samples,30–34 thus they can replace conventional organic dyes or quantum dots, and have evoked considerable interest for biological applications.
As molecular or ionic probes, UCNPs should combine with other chromophores through luminescence resonance energy-transfer (LRET) process. In a LRET system, UCNPs (donor) can transfer the energy to chromophores (acceptor), which will result in the change of upconversion luminescence (UCL). Nowadays, several LRET-based sensing and imaging systems have been reported. Li and co-workers developed cyanine-modified UCNPs for the detection of methylmercury,35 Liu and co-workers discovered manganese dioxide-loaded nanosheets for glutathione detection,36 and Chang and co-workers created dye-assembled UCNPs for sensing zinc ion in vitro and in vivo.37 Chen and co-workers used a sensitive time-resolved bioprobe to detect avidin through lanthanide-doped zirconia nanoparticles.38 Liu and co-workers demonstrated a NaYF4 sandwich structure for calcium ion detection39 and silver nanocluster modified UCNPs for biothiol detection.40 This year Qu and co-workers designed hyaluronic acid modified UCNPs for reactive oxygen species detection and bioimaging.41 Our group also reported progress on the detection of hydrogen peroxide,42 hypochlorous acid43 and hydrogen sulfide30 in living cells. Continuing our research in this direction, we hope to employ our method to detect mercury ions in biosystems because the mercury ion, a strong neurotoxin, accumulates in human bodies and causes severe nervous system damage.44 In the present study, we designed and fabricated an inorganic–organic hybrid probe for the detection of mercury ions. The as-prepared nanoprobe has excellent biological applications based on a LRET system that is composed of nanophosphors (NaYF4:20%Yb,1.8%Er,0.5%Tm) and a Hg2+-responsive thiazole derivative dye (Scheme 1). We chose hexagonal NaYF4 because of its higher upconversion efficiency, compared with its cubic counterpart.45 Using the ratio of UCL intensity at 540 nm to 803 nm (I540/I803) as the detection signal, we also demonstrated that the intensity of 540 nm would recover gradually with an increased amount of Hg2+. Moreover, the as-prepared UCNPs could monitor Hg2+ in living cells.
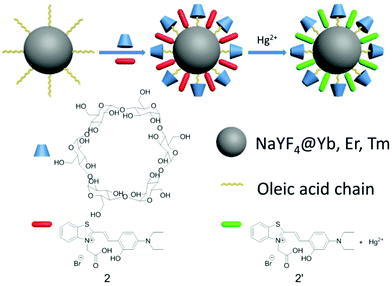 |
| Scheme 1 Schematic illustration of the structure design and proposed mechanism of Hg2+ detection. | |
Results and discussion
Design principle of the Hg2+ probe
Our design strategy was based on the fact that UCNPs could transfer energy to the chromophore by a LRET process (Scheme 2). To achieve this goal, the emission band of the UCNPs should overlap with the absorption band of the chromophore. Our target compound 2 has a maximum absorption peak at 546 nm, which perfectly matches the UCL emission of the 2H11/2 → 4I15/2 and 4S3/2 → 4I15/2 transitions of Er3+ (Fig. 1). As previously reported,46 the addition of Hg2+ to the solution of as-prepared nanoprobes will cause a blue-shift of the maximum absorption peak to 465 nm, forming 2′ and leading to the recovery of these UCL emission bands. Thus it is reasonable to suggest that the degree of energy transfer can be modulated by the concentration of Hg2+.
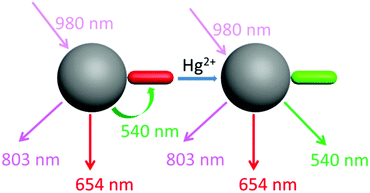 |
| Scheme 2 Schematic illustration of the LRET process from the UCNPs to compound 2. | |
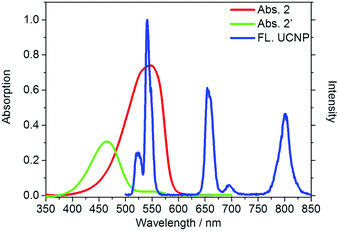 |
| Fig. 1 UV-Vis absorption (red and green) of compound 2 and 2′ and the upconversion emission (blue) of the OA-UCNPs. FL. UCNP refers to emission of OA-UCNP. | |
UCNPs are coated with oleic acids (OA), which are hydrophobic. In order to combine the UCNPs and compound 2 into one nanosystem, we employed α-cyclodextrin (α-CD) to convert the hydrophobic UCNPs into the hydrophilic form (Scheme 1) by self-assembly of host (α-CD) and guest molecules (OA).47,48 The surface of the as-converted nanoprobes was much easier for us to modify with compound 2, and improve the water solubility as well.
Synthesis and characterization of the UCNPs
Compound 2, containing a thiazole electron withdrawing group and a diethylamine electron donating group, was synthesized by a two-step reaction with an overall yield of 6.3% (Scheme 3).
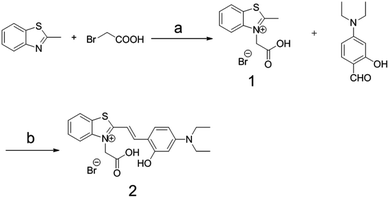 |
| Scheme 3 The synthesis route to compound 2. Reagents and conditions: (a) toluene, reflux, 20 h; (b) piperidine/ethanol, reflux, 24 h. | |
The OA-coated UCNPs were prepared by a modified solvothermal method.49–55 The as-prepared UCNPs were further functionalized by attaching to α-cyclodextrin,48 followed by loading compound 2 to produce three-layer nanostructure 2-UCNPs. Scheme 1 is a schematic illustration of UCNPs functionalized by the CD-capped OA ligands and compound 2. The hydrophilic parts of the hydroxyl groups face outwards, rendering the UCNPs with high water-solubility and stability.
Transmission electron microscopy (TEM) images (Fig. 2A–D) show that there are no significant changes in size, shape and crystallinity after modification with compound 2. These images also indicate that both OA-UCNPs and 2-UCNPs have an average diameter of about 25 nm, and no evident aggregation is observed. In addition, powder X-ray diffraction (PXRD) peaks of the UCNPs correlated very well with the hexagonal structure of NaYF4 (Fig. 2E), and all of the diffraction peaks of the 2-UCNPd could be indexed to the standard pattern of β-NaYF4, indicating the high purity of the 2-UCNPs. This result was further confirmed by high-resolution transmission electron microscopy (HR-TEM).
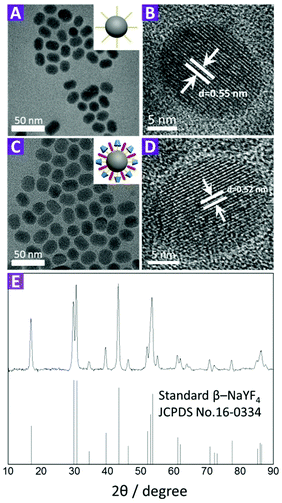 |
| Fig. 2 TEM (A) and HR-TEM (B) images of the OA-UCNPs. TEM (C) and HR-TEM (D) images of the 2-UCNPs. (E) XRD of the UCNPs (NaYF4:20%Yb,1.8%Er,0.5%Tm) and standard pattern of β-NaYF4 (JCPDS no. 16-0334). | |
The surface modification was also confirmed by Fourier-transform infrared (FTIR) spectroscopy (Fig. S6†). For OA-UCNPs, the band at 3433 cm−1 is attributed to the stretching vibration of the OH group, while two peaks at 2925 and 2854 cm−1 belong to both symmetric and asymmetric C–H stretching vibrations, indicating the presence of OA on the UCNP surfaces. For α-CD functionalized UCNPs, the band at 1077 cm−1 is attributed to C–O–C stretching vibration, confirming that α-CD has attached onto the UCNPs. Compared with the OA-UCNPs, the 2-UCNPs showed three new peaks at 1427, 1568, 1624 cm−1 and one new peak at 756 cm−1, which could be assigned to the C
C stretching vibration and C–H bending vibration of the benzene group of compound 2, respectively. These facts indicated that compound 2 was successfully assembled onto the surface of the UCNPs.
Sensing properties of compound 2
In the present study, we investigated the sensing ability of compound 2 for Hg2+ in DMSO/HEPES (1
:
9, v
:
v). The absorption spectra of compound 2 with and without Hg2+ are shown in Fig. S5.† In the absence of Hg2+, compound 2 had a maximum absorption peak at 546 nm (ε = 1.51 × 105 M−1 cm−1). With an increase in Hg2+ concentration, the peak at 546 nm gradually decreased, and the absorption peak at 465 nm increased with an isosbestic point at 481 nm, leading to an evident color change from red to green. This color change is attributed to the formation of a relatively stable metal complex.46,56,57 Both the sulfur atom in the benzothiazolium moiety and the oxygen atom in the phenolic moiety may combine with Hg2+, forcing the thiazole derivative to adopt a cis configuration, thus breaking the trans–cis dynamic equilibrium.
Sensing properties of the 2-UCNPs
After the dye-loading process, the sensing properties of the 2-UCNPs were also determined from UV-Vis absorption spectra and UCL spectra.
In the absorption spectrum, the 2-UCNPs showed a broad band with a maximum absorption at 540 nm. After the addition of Hg2+, the absorption peak was blue-shifted, changing from 540 nm to 463 nm, corresponding to a color change from red to green (Fig. 3A). This blue-shift (77 nm) was in agreement with the change of the pure compound 2, which indicated that a reaction between Hg2+ and compound 2 exists at the 2-UCNP surfaces. In addition, using A540/A463 as a detection signal, the limit of detection was measured to be 0.063 μM (Fig. S12†).
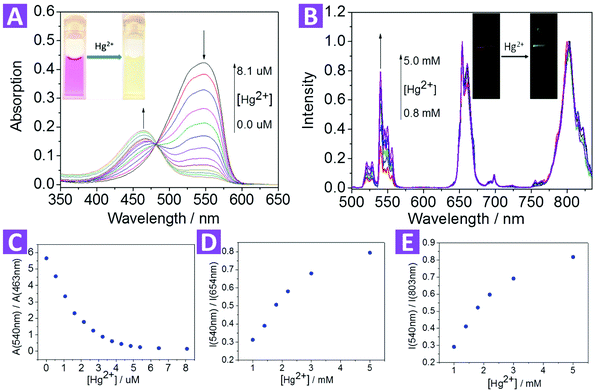 |
| Fig. 3 (A) Absorption spectrum of 0.005 mg mL−1 of 2-UCNPs in DMSO/HEPES (0.5 : 99.5, v : v) with different Hg2+ concentrations from 0–8.1 μM. Inset: the photo shows the color change from red to green. (B) UCL spectrum of 1 mg mL−1 of 2-UCNPs in DMSO with different Hg2+ concentrations from 0.8 to 5.0 mM. Inset: the photo shows the emission change from red to green. (C) The ratio of absorption at 540 nm to 463 nm decreased when the Hg2+ concentration increased. (D) The ratio of the UCL emission at 540 nm to 654 nm increased when the Hg2+ concentration increased. (E) The ratio of the UCL emission at 540 nm to 803 nm increased when the Hg2+ concentration increased. | |
As shown in Fig. 1, under excitation of 980 nm, the OA-UCNPs showed four UCL emission bands at 514–534 nm, 534–560 nm, 635–680 nm and 775–825 nm, attributed to the 2H11/2 → 4I15/2, 4S3/2 → 4I15/2 and 4F9/2 → 4I15/2 transitions of Er3+, and 3H4 → 3H6 of Tm3+, respectively. The dye-loaded UCNPs only showed 635–680 nm and 775–825 nm emission bands, and another two bands were almost quenched through the LRET process because they had overlapped with the absorption band of compound 2.
After the addition of Hg2+, the spectral overlap between the green emission band (514–560 nm) of the UCNPs and the absorption band (540 nm) of compound 2 was reduced, causing a decrease in the LRET process, thus the emission at 514–560 nm was recovered gradually (Fig. 3B). Note that another two emission bands at 635–680 nm and 775–825 nm were not involved in the LRET process, and their intensity was not affected before and after the addition of Hg2+, which means that these two bands could be used as a reference standard. Herein, we employed the ratio of the UCL intensity at 540 nm and 803 nm (I540/I803) as the detection signal, and the limit of detection was measured to be 0.21 μM (Fig. S13†). We also chose I540/I654 to improve the signal stability. As shown in Fig. 3D and E, the values of both I540/I803 and I540/I654 increased with the addition of Hg2+.
Compared with the 2-UCNPs, simply physically mixing the OA-UCNPs with compound 2 did not show obvious quenching of the 540 nm band. For the I540/I654 and I540/I803 values, physical mixing only led to a 4% and 8% decrease, respectively, indicating that the quenching effect of the 2-UCNPs was mainly ascribed to the LRET process. We tested the UCL intensity change after the physical mixing and found that the 540 nm band intensity decreased very fast (Fig. S8†). In 6 minutes at room temperature, the intensity decreased about 45% (Fig. S9†). These results indicated that compound 2 was easily combined with the UCNPs.
For an excellent ion probe, high selectivity plays a key role. To validate the selectivity of the 2-UCNPs, some other metal ions such as alkali (K+, Na+), alkali earth (Ca2+, Mg2+) and some transition metal ions (Mn2+) were tested under the same conditions for both absorption and emission (Fig. 4). For absorption, we calculated an absorbance ratio of 463 nm to 540 nm. Because the addition of Hg2+ would cause a significant decrease at 540 nm and an increase at 463 nm, the ratio of 463 nm to 540 nm would remarkably increase. Other metal ions did not show this effect, which led to small values of their absorption ratios. For the UCL spectrum, we calculated an intensity ratio of 540 nm to 803 nm. Hg2+ recovered the 540 nm band, increasing the ratio value, while the other ions did not. Compared with the absorption spectrum, the UCL intensity at 803 nm did not change, which means that the distinction between Hg2+ and the other ions seems to not be as obvious as in the absorption spectrum. In addition, to see if the existence of other ions would interfere with the detection of Hg2+, we tested the UCL intensity of the 2-UCNPs containing both Hg2+ and other ions (Fig. S10†). With or without other ions, the UCL intensity ratios only changed a little, indicating that the co-existence of other ions would not interfere with the detection of Hg2+.
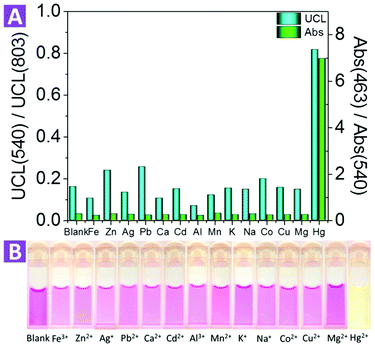 |
| Fig. 4 (A) The blue columns correspond to the ratio of the UCL emission at 540 nm to 803 nm of the 2-UCNPs (1 mg ml−1) with Hg2+ and other metal ions of same concentration (3.5 mmol L−1); the green columns correspond to the ratio of absorption at 463 nm to 540 nm of the 2-UCNPs (0.005 mg ml−1) with Hg2+ and other metal ions of the same concentration (20 μmol L−1). (B) Color change of the 2-UCNPs in the presence of different metal ions. | |
Furthermore, we also investigated the photo stability of the 2-UCNPs under exposure of 980 nm and 365 nm light. For 980 nm exposure, after 3 hours, the absorbance of the 2-UCNPs began to show a slight decrease. After 9 hours, the absorbance decreased by 2%. For 365 nm exposure, the absorbance had obviously decreased after 1 hour and showed a 27% decrease after 9 hours of illumination (Fig. S11†). Therefore, the nanosystem is more stable using UCL emission as a detection signal, and this significant improvement shows its potential for practical applications.
Monitoring the presence of mercury ions in living cells
Before the application of the 2-UCNPs in bioimaging, the cytotoxicity was investigated using the methyl thiazolyl tetrazolium (MTT) assay (Fig. S7†). Following the incubation of the 2-UCNPs for 24 h, the HeLa cells exhibited only minimal cytotoxicity. The cellular viability of the HeLa cells was still higher than 80% even at a high concentration of 800 μg mL−1, indicating that the 2-UCNPs form a biocompatible nanoprobe that is suitable for UCL bioimaging applications.
To demonstrate the applicability of the 2-UCNPs in monitoring intracellular Hg2+, we conducted a laser-scanning upconversion luminescence microscopy (LSUCLM) test (Fig. 5). Both the control (Fig. 5A–C) and test rows (Fig. 5D–F) were incubated with 0.5 mg mL−1 of 2-UCNPs for 180 minutes, and the test row was followed by incubated with 200 μM of Hg2+. Under 980 nm excitation, the control row cells only showed a weak UCL emission at 540 nm, indicating that the LRET process still happens in living cells. After the addition of Hg2+, an enhancement of the green emission was observed, which meant that the LRET process was blocked. Moreover, the intensity of the red emission hardly changed. As a result, there was an enhancement in the ratio of green to red emission. These results suggested that the 2-UCNPs could be used for monitoring intracellular Hg2+ through the ratiometric UCL method.
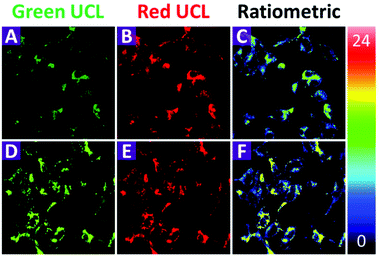 |
| Fig. 5 Ratiometric UCL images in HeLa cells (top, A to C) and 200 μM Hg2+ treated HeLa cells (bottom, D to F) incubated with 0.5 mg mL−1 of the 2-UCNPs for 180 minutes. Emission was collected by both the green channel at 500–560 nm (A and D) and red channel at 600–700 nm (B and E). (C and F) Ratiometric UCL images with the ratio of green to red channels. | |
Conclusion
In summary, we successfully modified the surface of UCNPs with compound 2 and demonstrated a new nanosystem for UCL detection and bioimaging of Hg2+ in living cells. The sensing mechanism was based on the blocking of the LRET process and recovery of the green emission band after the addition of Hg2+. We also employed the emission intensity ratio of 540 nm to 803 nm (I540/I803) as a signal to confirm that the green emission band increased with the addition of Hg2+. More importantly, this as-fabricated nanosystem was capable of monitoring Hg2+ in living cells, with low cytotoxicity. This method could provide a promising strategy for further detection and bioimaging probes.
Experimental section
Chemicals and materials
Column chromatography was conducted over silica gel (mesh 200–300). OA, 1-octadecane (ODE 90%), 2-methylbenzo[d]thiazole, 2-bromoacetic acid, 4-(diethylamino)-2-hydroxybenzaldehyde, α-cyclodextrin and NH4F were purchased from Sigma-Aldrich. YCl3·6H2O (99.9%), YbCl3·6H2O (99.9%), ErCl3·6H2O (99.9%), and TmCl3·6H2O (99.9%) were purchased from Alfa Aesar. Absolute ethanol, methanol, cyclohexane, dimethyl sulfoxide and methylene chloride were of analytical grade. All of the chemicals were used without further purification.
Instrumentation
1H-NMR was measured on a BrukerAV-400 spectrometer with chemical shifts reported in ppm (in CDCl3, CD3OD or DMSO-d6; TMS as an internal standard). Electrospray ionization mass spectrometry (ESI-MS) was carried out on a Micromass LCTTM system. X-ray diffraction was performed on a Shimadzu XRD-6000 diffractometer at a scanning rate of 1° min−1 with the 2θ range from 10 to 90° (Cu Kα radiation, λ = 1.54056 Å). HR-TEM was carried out on a JEOL JEM-2100F transmission electron microscope with an accelerating voltage of 200 kV. UV–Vis spectra were recorded by a Shimadzu UV-2501 spectrometer. The UCL spectra were obtained by a DM150i monochromator equipped with a R928 photon counting photomultiplier tube (PMT), in conjunction with a 980 nm diode laser. FTIR spectra were obtained using a Perkin-Elmer Lambda 783 spectrometer with KBr pellets.
Synthesis of Hg2+ probe
Compound 1.
2-Methylbenzo[d]thiazole (1.511 g, 10.1 mmol), 2-bromoacetic acid (2.184 g, 15.7 mmol) and 50 ml of toluene were added into a one-neck flask. After refluxing for 20 hours, the solution was cooled down and the resultant precipitate was filtered and washed four times with 2 ml of methanol/methylene chloride (1
:
9, v
:
v) to give a light grey solid (0.358 g): yield 12.2%; 1H NMR (400 MHz, CDCl3, TMS): δ = 3.18 (s, 3H, Me), 5.74 (s, 2H, NCH2), 7.79–7.83 (m, 1H, Ph), 7.87–7.91 (m, 1H, Ph), 8.27 (d, 1H, Ph), 8.45 (d, 1H, Ph).
Compound 2.
Compound 1 (0.337 g, 1.17 mmol) and 4-(diethylamino)-2-hydroxybenzaldehyde (0.231 g, 1.20 mmol) were dissolved in 40 ml of ethanol, and 40 μl of piperidine was added as a catalyst. After refluxing for 24 hours, the mixture was cooled down and the resulting precipitate was filtered and washed with 2 ml of methylene chloride/ethyl ether (1
:
9, v
:
v) mixture for four times to produce a dark green solid (280 mg): yield 51.7%; 1H NMR (400 MHz, CD3OD, TMS): δ = 1.23 (t, 6H, Me), 3.45–3.52 (m, 4H, NCH2), 5.24 (s, 2H, NCH2), 6.14 (d, 1H, Ph), 6.40–6.44 (d, 1H, Ph), 7.35 (d, 1H, Ph), 7.54 (m, 2H, Ph), 7.59 (t, 1H, Ph), 7.67 (d, 1H, Ph), 8.00 (d, 1H, Ph), 8.20 (d, 1H, Ph). MS: calculated for C21H23N2O3S+ 383.14, found 383.29. ESI-MS: m/z 383.29.
Synthesis of the OA-UCNPs
The OA-UCNPs were synthesized by a modified procedure according to a previous report.49–55 YCl3·6H2O (235.7 mg, 0.777 mmol), YbCl3·6H2O (77.5 mg, 0.20 mmol), ErCl3·6H2O (6.9 mg, 0.018 mmol) and TmCl3·6H2O (1.9 mg, 0.005 mmol) were dissolved in 10 ml of methanol by sonication. After removing the methanol, 7 ml of oleic acid and 15 ml of 1-octadecene were added. The mixture was heated up to 160 °C for 30 minutes and a homogeneous solution was formed. After cooling to room temperature, 10 ml of methanol solution containing NaOH (100 mg, 2.5 mmol) and NH4F (148 mg, 4 mmol) were added. Under an argon environment, the resulting colloidal mixture was slowly heated up to 140 °C for 10 minutes to remove the methanol, and then the heating was increased to 305 °C, and maintained for 1.5 hours. After cooling down the solution naturally, the nanoparticles were obtained by adding ethanol, followed by centrifugation and were washed with ethanol three times.
Synthesis of α-CD functionalized UCNPs
CD-UCNPs were synthesized by a modified procedure.48 12 ml of ethanol/water (2
:
1, v
:
v) solution containing 60 mg of the OA-UCNPs was mixed with 12 ml of an α-cyclodextrin (α-CD) aqueous solution (20 mg ml−1). After vigorously stirring the mixture at room temperature, a transparent solution resulted. After stirring for 20 hours at room temperature, the solution was centrifuged (10
000 rpm, 20 minutes) and the resulting particles were washed with deionized water three times.
Synthesis of the 2-UCNPs
20 mg of compound 2 was dissolved in 4 ml of ethanol, and mixed with 10 mg of the α-CD functionalized UCNPs. After heating at 70 °C for 24 hours, the mixture was cooled down and the solution was centrifuged (9000 rpm, 10 minutes). The as-obtained particles were washed with ethanol three times and dried in a fume hood.
Cytotoxicity assay
To verify the cytotoxic effect of the 2-UCNPs, an MTT assay was performed by treating the HeLa cells with a 24 h incubation. Cells were passed and plated to a 70% confluence in 96-well plates, and cultured in growth medium at 37 °C and 5% CO2 for 24 h. Different doses of the 2-UCNPs (100, 200, 500, and 800 μg mL−1) were added to the HeLa cells under the same conditions, followed by incubation with 5 mg mL−1 of MTT reagent for 4 h and the absorbance of each well was measured by a microplate reader (SPECTRA SLT; Lab instruments, Salzburg, Austria). Each treatment was done in six wells, and the experiments were repeated twice. Cytotoxicity was calculated relative to the absorbance of the control for each treatment. The reported percentage of cell survival values were relative to untreated control cells.
Acknowledgements
Q. Z. acknowledges financial support from AcRF Tier 1 (RG 16/12 and RG133/14) and Tier 2 (ARC 20/12 and ARC 2/13) from MOE, and the CREATE program (Nanomaterials for Energy and Water Management) from NRF, Singapore. Q. Z. also thanks the support from the Open Project of State Key Laboratory of Supramolecular Structure and Materials (Grant Number: sklssm2015027), Jilin University, China.
Notes and references
- Y. Yin and A. P. Alivisatos, Nature, 2005, 437, 664–670 CrossRef CAS PubMed
.
- H. R. Herschman, Science, 2003, 302, 605–608 CrossRef CAS PubMed
.
- V. Sokolova and M. Epple, Angew. Chem., Int. Ed., 2008, 47, 1382–1395 CrossRef CAS PubMed
.
- K.-T. Yong, I. Roy, M. T. Swihart and P. N. Prasad, J. Mater. Chem., 2009, 19, 4655–4672 RSC
.
- Y. Wang, Z. Chen, Y. Liu and J. Li, Nanoscale, 2013, 5, 7349–7355 RSC
.
- Z. Wang, Z. Yan, N. Sun and Y. Liu, Biosens. Bioelectron., 2015, 68, 771–776 CrossRef CAS PubMed
.
- Y. Wang, K. Qu, L. Tang, Z. Li, E. Moore, X. Zeng, Y. Liu and J. Li, TrAC, Trends Anal. Chem., 2014, 58, 54–70 CrossRef CAS
.
- X. Chen, Y. Wang, Y. Zhang, Z. Chen, Y. Liu, Z. Li and J. Li, Anal. Chem., 2014, 86, 4278–4286 CrossRef CAS PubMed
.
- J.-C. G. Buenzli, Chem. Rev., 2010, 110, 2729–2755 CrossRef CAS PubMed
.
- A. Y. Louie, Chem. Rev., 2010, 110, 3146–3195 CrossRef CAS PubMed
.
- Q. Ju, D. Tu, Y. Liu, H. Zhu and X. Chen, Comb. Chem. High Throughput Screening, 2012, 15, 580–594 CrossRef CAS PubMed
.
- K. E. Sapsford, W. R. Algar, L. Berti, K. B. Gemmill, B. J. Casey, E. Oh, M. H. Stewart and I. L. Medintz, Chem. Rev., 2013, 113, 1904–2074 CrossRef CAS PubMed
.
- M. S. T. Gonçalves, Chem. Rev., 2009, 109, 190–212 CrossRef PubMed
.
- Y. Xing and J. Rao, Cancer Biomark., 2008, 4, 307–319 CAS
.
- E. Downing, L. Hesselink, J. Ralston and R. Macfarlane, Science, 1996, 273, 1185–1189 CAS
.
- F. Wang and X. Liu, J. Am. Chem. Soc., 2008, 130, 5642–5643 CrossRef CAS PubMed
.
- F. Wang, X. Xue and X. Liu, Angew. Chem., Int. Ed., 2008, 47, 906–909 CrossRef CAS PubMed
.
- F. Auzel, Chem. Rev., 2004, 104, 139–174 CrossRef CAS PubMed
.
- J. Zhou, Z. Liu and F. Y. Li, Chem. Soc. Rev., 2012, 41, 1323–1349 RSC
.
- M. Haase and H. Schäfer, Angew. Chem., Int. Ed., 2011, 50, 5808–5829 CrossRef CAS PubMed
.
- M. Yu, F. Li, Z. Chen, H. Hu, C. Zhan, H. Yang and C. Huang, Anal. Chem., 2009, 81, 930–935 CrossRef CAS PubMed
.
- H. Zijlmans, J. Bonnet, J. Burton, K. Kardos, T. Vail, R. S. Niedbala and H. J. Tanke, Anal. Biochem., 1999, 267, 30–36 CrossRef CAS PubMed
.
- L. Xiong, Z. Chen, Q. Tian, T. Cao, C. Xu and F. Li, Anal. Chem., 2009, 81, 8687–8694 CrossRef CAS PubMed
.
- F. Wang, Y. Han, C. S. Lim, Y. Lu, J. Wang, J. Xu, H. Chen, C. Zhang, M. Hong and X. Liu, Nature, 2010, 463, 1061–1065 CrossRef CAS PubMed
.
- J. Zhou, Y. Sun, X. Du, L. Xiong, H. Hu and F. Li, Biomaterials, 2010, 31, 3287–3295 CrossRef CAS PubMed
.
- N. M. Idris, Z. Li, L. Ye, E. K. Wei Sim, R. Mahendran, P. C.-L. Ho and Y. Zhang, Biomaterials, 2009, 30, 5104–5113 CrossRef CAS PubMed
.
- Z. Zhou, H. Hu, H. Yang, T. Yi, K. Huang, M. Yu, F. Li and C. Huang, Chem. Commun., 2008, 4786–4788, 10.1039/b809021a
.
- Z. Chen, H. Chen, H. Hu, M. Yu, F. Li, Q. Zhang, Z. Zhou, T. Yi and C. Huang, J. Am. Chem. Soc., 2008, 130, 3023–3029 CrossRef CAS PubMed
.
- Y. I. Park, K. T. Lee, Y. D. Suh and T. Hyeon, Chem. Soc. Rev., 2015, 44, 1302–1317 RSC
.
- Y. Zhou, W. Chen, J. Zhu, W. Pei, C. Wang, L. Huang, C. Yao, Q. Yan, W. Huang, J. S. C. Loo and Q. Zhang, Small, 2014, 10, 4874–4885 CrossRef CAS PubMed
.
- C. Wang, L. Cheng and Z. Liu, Biomaterials, 2011, 32, 1110–1120 CrossRef CAS PubMed
.
- J.-C. Zhou, Z.-L. Yang, W. Dong, R.-J. Tang, L.-D. Sun and C.-H. Yan, Biomaterials, 2011, 32, 9059–9067 CrossRef CAS PubMed
.
- L. Xiong, T. Yang, Y. Yang, C. Xu and F. Li, Biomaterials, 2010, 31, 7078–7085 CrossRef CAS PubMed
.
- H. Hu, M. Yu, F. Li, Z. Chen, X. Gao, L. Xiong and C. Huang, Chem. Mater., 2008, 20, 7003–7009 CrossRef CAS
.
- Y. Liu, M. Chen, T. Cao, Y. Sun, C. Li, Q. Liu, T. Yang, L. Yao, W. Feng and F. Li, J. Am. Chem. Soc., 2013, 135, 9869–9876 CrossRef CAS PubMed
.
- R. Deng, X. Xie, M. Vendrell, Y.-T. Chang and X. Liu, J. Am. Chem. Soc., 2011, 133, 20168–20171 CrossRef CAS PubMed
.
- J. Peng, W. Xu, C. L. Teoh, S. Han, B. Kim, A. Samanta, J. C. Er, L. Wang, L. Yuan, X. Liu and Y.-T. Chang, J. Am. Chem. Soc., 2015, 137, 2336–2342 CrossRef CAS PubMed
.
- Y. Liu, S. Zhou, D. Tu, Z. Chen, M. Huang, H. Zhu, E. Ma and X. Chen, J. Am. Chem. Soc., 2012, 134, 15083–15090 CrossRef CAS PubMed
.
- Z. Li, S. Lv, Y. Wang, S. Chen and Z. Liu, J. Am. Chem. Soc., 2015, 137, 3421–3427 CrossRef CAS PubMed
.
- Y. Xiao, L. Zeng, T. Xia, Z. Wu and Z. Liu, Angew. Chem., Int. Ed., 2015, 54, 5323–5327 CrossRef CAS PubMed
.
- Z. W. Chen, Z. Liu, Z. H. Li, E. G. Ju, N. Gao, L. Zhou, J. S. Ren and X. G. Qu, Biomaterials, 2015, 39, 15–22 CrossRef CAS PubMed
.
- Y. Zhou, W. Pei, X. Zhang, W. Chen, J. Wu, C. Yao, L. Huang, H. Zhang, W. Huang, J. S. Chye Loo and Q. Zhang, Biomaterials, 2015, 54, 34–43 CrossRef CAS PubMed
.
- Y. Zhou, W. Pei, C. Wang, J. Zhu, J. Wu, Q. Yan, L. Huang, W. Huang, C. Yao, J. S. C. Loo and Q. Zhang, Small, 2014, 10, 3560–3567 CrossRef CAS PubMed
.
- Z. Guo, W. Zhu, M. Zhu, X. Wu and H. Tian, Chem. – Eur. J., 2010, 16, 14424–14432 CrossRef CAS PubMed
.
- K. W. Kramer, D. Biner, G. Frei, H. U. Gudel, M. P. Hehlen and S. R. Luthi, Chem. Mater., 2004, 16, 1244–1251 CrossRef
.
- S. Tatay, P. Gaviña, E. Coronado and E. Palomares, Org. Lett., 2006, 8, 3857–3860 CrossRef CAS PubMed
.
- Q. Liu, M. Chen, Y. Sun, G. Chen, T. Yang, Y. Gao, X. Zhang and F. Li, Biomaterials, 2011, 32, 8243–8253 CrossRef CAS PubMed
.
- Y. Wang, J. F. Wong, X. Teng, X. Z. Lin and H. Yang, Nano Lett., 2003, 3, 1555–1559 CrossRef CAS
.
- Z. Li, Y. Zhang and S. Jiang, Adv. Mater., 2008, 20, 4765–4769 CrossRef CAS
.
- H.-X. Mai, Y.-W. Zhang, R. Si, Z.-G. Yan, L.-d. Sun, L.-P. You and C.-H. Yan, J. Am. Chem. Soc., 2006, 128, 6426–6436 CrossRef CAS PubMed
.
- X. Wang, J. Zhuang, Q. Peng and Y. Li, Nature, 2005, 437, 121–124 CrossRef CAS PubMed
.
- Q. Liu, W. Feng, T. Yang, T. Yi and F. Li, Nat. Protoc., 2013, 8, 2033–2044 CrossRef CAS PubMed
.
- F. Wang, R. Deng and X. Liu, Nat. Protoc., 2014, 9, 1634–1644 CrossRef CAS PubMed
.
- C. Li, Z. Quan, J. Yang, P. Yang and J. Lin, Inorg. Chem., 2007, 46, 6329–6337 CrossRef CAS PubMed
.
- H.-S. Qian and Y. Zhang, Langmuir, 2008, 24, 12123–12125 CrossRef CAS PubMed
.
- J. T. C. Wojtyk, P. M. Kazmaier and E. Buncel, Chem. Mater., 2001, 13, 2547–2551 CrossRef CAS
.
- O. A. Fedorova, Y. V. Fedorov, A. I. Vedernikov, S. P. Gromov, O. V. Yescheulova, M. V. Alfimov, M. Woerner, S. Bossmann, A. Braun and J. Saltiel, J. Phys. Chem. A, 2002, 106, 6213–6222 CrossRef CAS
.
Footnote |
† Electronic supplementary information (ESI) available: NMR, MALDI-TOF MS spectra, etc. See DOI: 10.1039/c5nr05286f |
|
This journal is © The Royal Society of Chemistry 2016 |
Click here to see how this site uses Cookies. View our privacy policy here.