DOI:
10.1039/C5RA24781K
(Paper)
RSC Adv., 2016,
6, 4116-4127
Controllable synthesis of rod-like SnO2 nanoparticles with tunable length anchored onto graphene nanosheets for improved lithium storage capability
Received
23rd November 2015
, Accepted 22nd December 2015
First published on 28th December 2015
Abstract
In our work, rod-like SnO2 nanoparticles with tunable length have been successfully anchored onto graphene nanosheets through a simple and in situ hydrothermal strategy under acidic conditions. The SEM and TEM images demonstrate that the unique rod-like SnO2 nanoparticles with a diameter of 10–15 nm and length of 18–34 nm are uniformly anchored onto the surface of graphene nanosheets. Moreover, the particle sizes of rod-like SnO2 nanoparticles can be readily adjusted by simply varying the reaction temperature. Interestingly, with the increase of reaction temperature from 120 to 160 °C, the rod length of SnO2 nanoparticles significantly increased. More importantly, the SnO2@graphene products exhibit a very high specific surface area, which played a key role in maintaining the structural stability against the irreversible volume change during Li+ insertion/extraction. The nanocomposites show an extremely high lithium storage capability and an excellent cycling performance. The initial discharge capacities are 1284 mA h g−1 at current densities of 200 mA g−1. After 100 cycles, the discharge capacity still remains as high as 981 mA h g−1, indicating a superior retention capacity.
1. Introduction
As the dominant power source, rechargeable lithium-ion batteries (LIBs) have been extensively applied in various fields because of their well-known advantages including high energy intensity, long cycle life and no memory effect. However, the current LIBs technology can't meet the ever-growing demands for large-scale applications such as electric vehicles and scalable electricity storage. Consequently, advanced electrode materials with high energy density, long cycle life, and good rate performances are highly desirable.1–5 Among the potential anode materials for LIBs, a lot of metals, metal oxides, and metal sulfides have attracted more and more attention because their theoretical capacities are higher than the commercial graphite anodes (372 mA h g−1). In the case, various materials with different morphologies have been thoroughly developed for a wide range of applications such as zero-dimensional (0D) nanoparticle,6,7 one dimensional (1D) nanorod/nanofibre/nanotube,8,9 two dimensional (2D) nanosheet/nanoplate,10,11 and three dimensional (3D) architectures.12 Particularly, SnO2 nanomaterial has been regarded as one of the most promising candidates for alternative anodes of Li-ion batteries due to its high theoretical gravimetric lithium storage capacity of 782 mA h g−1 and low potential of lithium-ion intercalation.13,14 Nevertheless, the practical application of SnO2 anodes is still restricted due to their large volume change (200–300%)15 and the bad aggregation during cycles, resulting in a rapid capacity fading and short cycle life.16 Therefore, much attention should be paid to explore suitable electrode materials with good electrochemical cyclic stability and simple fabrication techniques.
Graphene, as a sparkling star, has been served as an intriguing substrate to build up 2D nanohybrids for energy storage and conversions,17–19 owing to its two dimensional (2D) structure,20,21 high specific surface area,22 excellent charge carrier mobility23 and good mechanical properties.24 Firstly, the graphene nanosheets can provide an elastic buffer space to accommodate the volume expansion/contraction of the supported materials during the Li insertion/extraction process. Secondly, the 2D structure of graphene can act as a support to immobilize the supported materials upon repetitive cycling and prevent their agglomeration. Furthermore, the porosity and defects generated in the composite can increase the electrode/electrolyte contact area and provide more active sites for lithium reaction and storage.25 Hence, the excellent properties could effectively improve the electrochemical performances during cycling. Despite the great progress has been made for the construction of SnO2@graphene composites with versatile nanostructures including nanoparticles, nanosheets, nanoflowers and nanospheres.26–30 Nevertheless, the destruction of the desirable morphologies for electrodes could not be truly avoided especially at long-term cycles and high rates.
Herein, we present an environment-friendly, one-pot and in situ hydrothermal route for fabrication of SnO2@graphene nanocomposites starting from SnCl2·2H2O and graphene with the assistance of cetyltrimethylammoniumbromide (CTAB). The structure and composition of the products are analyzed in detail by SEM, TEM, XRD, and BET techniques. The resulting products show that the rod-like SnO2 nanoparticles are homogeneously anchored onto the graphene matrix. The excellent electrochemical performance is mainly attributed to the conducting, buffering and protective effects of graphene nanosheets onto the rod-like SnO2 nanoparticles, as well as the uniform morphology and high specific surface area. We expect that the prepared materials will provide more potential applications in various fields including the dye-sensitized solar cell, sensor and optical devices.
2. Experimental section
2.1 Materials
All the chemical reagents in the experiments were used without further purification. Stannous chloride dihydrate (SnCl2·2H2O), natural graphite powder, phosphorus-pentoxide (P2O5), potassium hypermanganate (KMnO4), hydrogen-peroxide (H2O2), potassium persulfate (K2S2O8), sulfuric acid (H2SO4), cetyltrimethylammonium bromide (CTAB), hydrochloric acid (HCl, 37 wt%) and ethanol were purchased from Sinopharm Chemical Reagent Co., Ltd.
2.2 Synthesis of SnO2@graphene nanocomposites
Graphene oxide (GO) sheets were synthesized from natural graphite powder by a modified Hummers' method.31 Here, the reduced graphene oxide (RGO) was further obtained by a thermal reduction of the as-synthesized GO. The details was described in a previous work.32 SnO2@graphene nanocomposites were prepared by a simple hydrothermal route. In a typical synthesis, 50 mg of graphene nanosheets (RGO) was dispersed into 60 mL of ethanol/water (2
:
4, in volume) mixed solutions by ultrasonication for 2 h to get a suspension. Then, 0.55 g of cetyltrimethylammonium bromide (CTAB) and 2 mL of HCl solution (37 wt%) were added the above suspension under stirring for 30 min. Afterwards, 50 mg of SnCl2·2H2O was added to the above mixture with magnetic stirring for 1 h. Subsequently, the mixture was transferred to 150 mL Teflon-lined stainless steel autoclave and maintained at 120–160 °C for 24 h. After cooling to room temperature naturally, the precipitate was collected by centrifuge and repeatedly washed with ethanol and deionized water, and then dried at 60 °C overnight. The resultant composites were obtained, in which the SnO2@graphene was denoted as the SG-120, SG-140, and SG-160, respectively, corresponding to the hydrothermal reaction temperate. For comparison, pure SnO2 nanoparticles were also prepared according to our previous publication work.33
2.3 Characterizations
X-ray powder diffraction (XRD) patterns were obtained on the Japan Rigaku D/max-2550 instrument using CuKα radiation. FT-IR spectrum was recorded on a Nicolet AVATAR 370 Fourier transform infrared spectrometer using KBr pellets. Raman spectra were collected using a Renishaw inVia system with an excitation wavelength of 514 nm. The structure and morphology of the samples were investigated by transmission electron microscopy (TEM, JEOL 200CX), scanning electron microscopy (SEM, JEOL JSM-6700F), and high resolution transmission electron microscopy (HRTEM, JSM-2010F). Elemental qualitative analysis was performed by the energy-dispersive X-ray spectroscopy (EDX, OXFORD INCA). X-ray photoelectron spectra (XPS) were detected with a Kratos Analytical Axis UltraDLD spectrometer with Mg Kα X-ray radiation. The C1s line (284.6 eV) was taken as a reference to calibrate the shift of binding energy due to electrostatic charging. N2 sorption isotherms were measured on a QUADRASORB SI surface area & pore size analyzer at 77 K, after the products were degassed in vacuum at 150 °C for 6 h. The Brunauer–Emmett–Teller (BET) specific surface area and the pore size distribution were calculated by using the desorption data. Thermogravimetric (TG) analysis was provided with a Netzsch TG 209F1 system in a flow of air.
2.4 Electrochemical measurements
The working electrodes were fabricated as follows: 80 wt% of the active material (SG-120, SG-140, SG-160, and pure SnO2, respectively), 10 wt% of the conductive agent (acetylene black), and 10 wt% of the binder (poly(vinylidene difluoride), PVDF) were homogeneously mixed in N-methyl-2-pyrrolidinone (NMP) and pasted on a copper foil. The electrochemical properties of the working electrodes were measured using two electrodes CR2016 coin-type cells with lithium foil serving as both counter and reference electrodes under ambient temperature. The electrolyte was 1 M LiPF6 solution in EC/DMC/EMC (ethylene carbonate/dimethyl carbonate/ethyl methyl carbonate, 1/1/1, w/w/w, Zhangjiagang Guotai-Huarong New Chemical Materials Co., Ltd). The galvanostatic discharge-charge tests were carried out using a LAND-CT2001A test system. The coin cells were discharged and charged at different current densities (50–2000 mA g−1) in the fixed voltage range of 0–3.0 V. The first cycle discharging was kept at 50 mA g−1. The electrochemical measurement of pure graphene sheets (RGO) was followed the same procedure except without addition of carbon black, in which the weight ratio of graphene sheets to PTFE was at 80
:
20.
2.5 Structure and morphology
As shown in Scheme 1, the SnO2@graphene nanocomposites are prepared by a simple hydrothermal method, in which the length of SnO2 nanoparticles decorated into the graphene can be well adjusted by easily altering the reaction temperature. Firstly, GO nanosheets with abundant functional groups (e.g. carboxyl and hydroxyl) are prepared through the modified Hummers method. Subsequently, the reduced GO nanosheets (RGO) are obtained by annealing GO at 500 °C for 2 h in nitrogen atmosphere and the prepared RGO nanosheets are uniformly dispersed in the ethanol/water solutions. Afterwards, SnCl2·2H2O is added to the CTAB/HCl mixed solutions under stirring, in which Sn2+ ions are hydrolyzed to SnO2 nanoparticles and firmly attach onto the surface of graphene nanosheets. At the end, the rod-like SnO2 nanoparticles are uniformly dispersed onto the surface of RGO. During the process, the defects in RGO serve as docks in favor of Sn2+ ions to be adsorbed on the surface of graphene. It should be noted that the size of SnO2 nanoparticles could be easily tuned by only changing the reaction temperature.
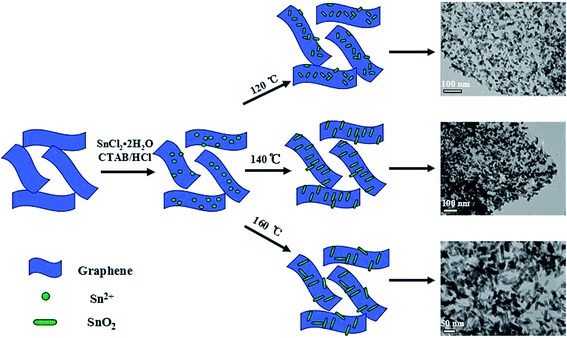 |
| Scheme 1 Schematic illustration for the formation of rod-like SnO2@graphene nanocomposites. | |
The morphology and structure of SnO2@graphene nanocomposites are examined by electron microscopy technique. TEM images (Fig. 1(a), (d) and (g)) reveal that a lot of uniform SnO2 nanoparticles have been deposited onto the high quality graphene substrate, regardless of the reaction temperature. No free particles or unloaded graphene sheets could be obviously seen. The magnified TEM images demonstrate that the nanoparticles are rod-like morphology with a diameter of 10–15 nm and a length of 18–34 nm (Fig. 1(b), (e) and (h)). Interestingly, with the increase of reaction temperature from 120 to 160 °C, the rod length of SnO2 nanoparticles significantly increases. However, the change of the diameter is very little. The particle size distributions further confirm the fact, in which the length of the SnO2 nanoparticles is 18.2, 28.8, 33.8 nm, respectively, but little change in diameter, about 10.5, 13.9, 14.2 nm (Fig. 1(c), (f) and (i)) corresponding to the reaction temperature from 120 to 160 °C. Usually, a crystal prefers to expose crystal planes with low surface energy in the growth process. For example, both natural and synthetic forms of SnO2 crystals are generally enclosed by (110), (101), or (100) facets with low surface energy.34 In present SnO2@graphene nanocomposite, the crystal growth of the rod-like SnO2 nanoparticles seems to also follow the trend. In addition, it should be mentioned that without CTAB in the precursor, some SnO2 nanoparticles are not fully loaded onto the RGO (not shown). As a result, it can be concluded that the addition of CTAB played a key role for the formation of the desired SnO2@graphene nanocomposites. Certainly, the reaction temperature is also a very important parameter.
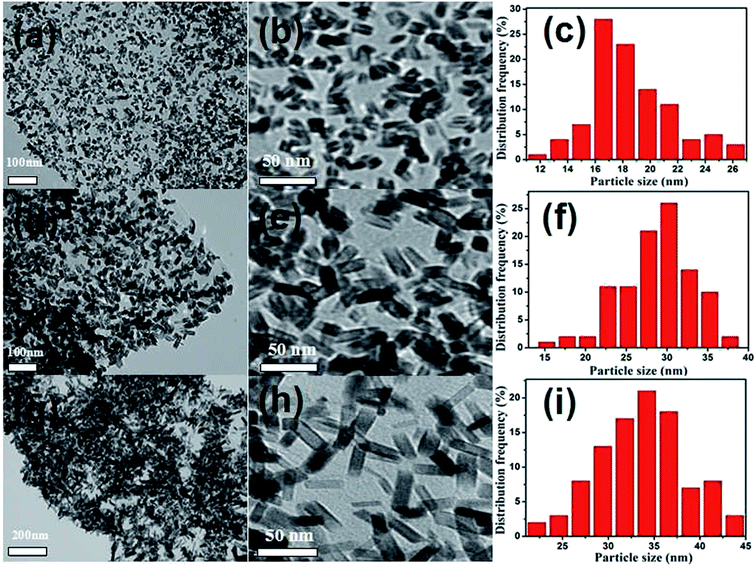 |
| Fig. 1 TEM images with different magnification and the corresponding particle size distributions of various SnO2@graphene nanocomposites: (a–c) SG-120, (d–f) SG-140, (g–i) SG-160. | |
Fig. 2 shows the low-magnification SEM images and the corresponding EDX patterns of various SnO2@graphene nanocomposites. Seen from the pictures (Fig. 2(a), (c) and (e)), all products synthesized at the range of 120–160 °C have homogeneous morphology, in which a number of SnO2 nanoparticles grow onto the graphene matrix, in agreement with the TEM analysis. Only Sn, C and O elements are detected in the corresponding EDX patterns (Fig. 2(b), (d) and (f)), no matter the reaction temperature, suggesting a high purity of all products. While a small amount of Si element is observed, origining from the Si substrate used to disperse SEM samples. Meanwhile, that will avoid the carbon effect from the common carbon substrate.
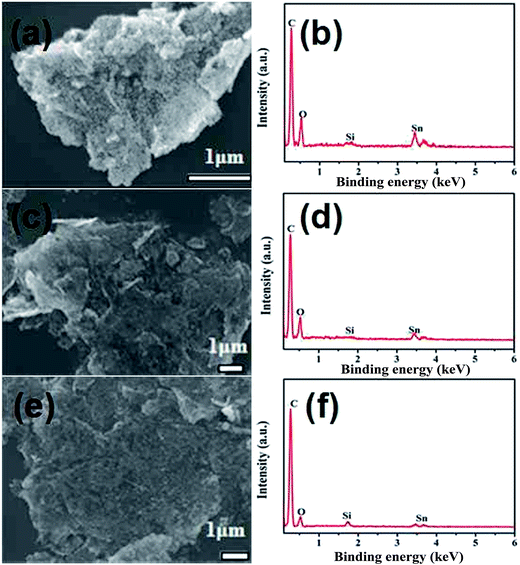 |
| Fig. 2 SEM images and the corresponding EDX patterns of various SnO2@graphene nanocomposites: (a and b) SG-120, (c and d) SG-140, (e and f) SG-160. | |
The crystal structure of SnO2@graphene nanocomposites is investigated by XRD technique. As can be seen from the Fig. 3, an obvious diffraction peak at 2θ = 10.3° in GO nanosheet is observed, owing to the (001) crystal plane of GO, indicating that GO has been successfully obtained via the chemical oxidation of graphite powder. The absence of this peak in the SnO2@graphene nanocomposites indicates that the SnO2 nanoparticles effectively inhibit the re-stacking of graphene nanosheets, in other words, that limits constructive diffractions from ordered graphene sheets, indirectly confirming the formation of SnO2@graphene nanocomposites. The four main diffraction peaks corresponding to the (110), (101), (211), and (310) are attributed to the tetragonal SnO2 with cassiterite structure (JCPDS card no. 41-1445).35,36 Compared with bare SnO2 nanoparticle, the intensity of the diffraction peaks in the all SnO2@graphene nanocomposites is lower and wider. That suggests the influence of thermal reduction of graphene oxide on the growth of SnO2 nanoparticles.
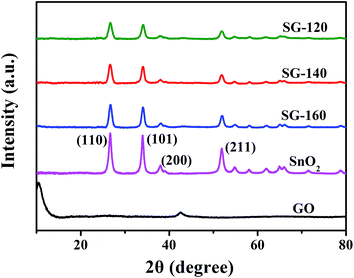 |
| Fig. 3 XRD patterns of various products. | |
Fig. 4(a) shows the FT-IR spectra of SnO2@graphene nanocomposites. All samples display strong absorption assigned to the carbon oxygen bonds from GO in the wavenumber region of 900–1800 cm−1.37 The peaks at 1721 cm−1 and 1680 cm−1 are due to the characteristic absorption of –COOH and C
C, respectively.38 The broad absorption band located at 3421 cm−1 is ascribed to the stretching vibration of –OH. However, the characteristic peaks of GO in SnO2@graphene become weaker at the wavenumber region of 900–1800 cm−1. The absorption located at 1721 cm−1 even disappears.39,40 These results demonstrate the successful reduction of the oxygen-containing functional groups of GO during the hydrothermal treatment. The broad absorption band located at 673 cm−1 comes from the stretching vibration of O–Sn–O, which can be seen in the three SnO2@graphene nanocomposites.32
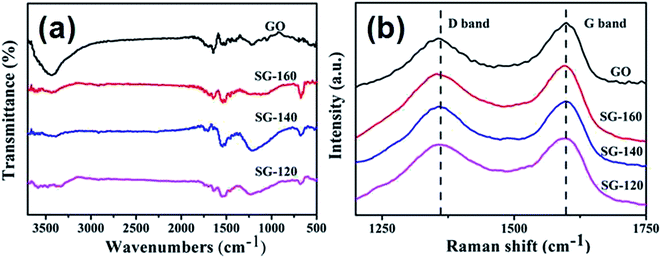 |
| Fig. 4 (a) FT-IR spectra, (b) Raman spectra of various products. | |
Raman spectra are further characterized the carbon framework. As illustrated in Fig. 4(b), the peak at 1590 cm−1 (G band) in the Raman spectra of the SnO2@graphene composites is usually assigned to the E2g phonon of C sp2 atoms, while the peak at 1350 cm−1 (D band) is the breathing mode of k-point phonons of A1g symmetry.41,42 The intensity ratio (ID/IG) of D band to G band is related to the extent of p-conjugation and concentration of defects, which is 0.78, 0.93, 0.95 and 0.92, respectively, for GO, SG-120, SG-140 and SG-160. The difference in ID/IG value reveals the electronic interactions between rod-like SnO2 nanoparticles and graphene substrate, which is beneficial to electron transport between SnO2 and graphene and the formation of the three-dimensional network, thus also desirable for lithium storage.
N2 adsorption–desorption isotherm analysis is employed to further investigate the textural properties of the products. As presented in Fig. 5, all samples are of type IV isotherm curves with a distinct hysteresis loop at the relatively pressure p/p0 = 0.4–1.0, suggesting their mesoporous structures. And all products exhibit the high specific surface areas. The specific surface area of SG-140 is calculated to be 544.2 m2 g−1, much higher than that of SG-160 (511.6 m2 g−1), lower than of that of SG-120 (610.5 m2 g−1). The high specific surface area should be mainly attributed to the contribution of graphene architecture. The corresponding pore size distribution plots calculated from the desorption isotherm using the Barrett–Joyner–Halenda (BJH) method are shown in Fig. 5(b), (d) and (f). The results show that the three samples all possess small mesoporous mainly peaked at 3.9 nm, which is mainly ascribed to interparticle aggregation of adjacent SnO2 nanoparticles. As a consequence, such a high specific surface area and narrow pore size distribution in SnO2@graphene nanocomposites are expected to exhibit superior performance as anode materials for lithium-ion storage.
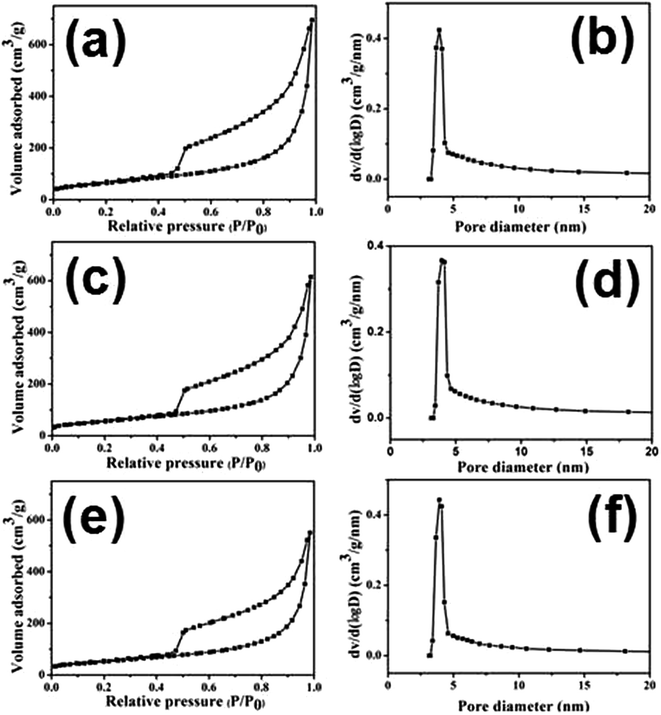 |
| Fig. 5 N2 adsorption–desorption isotherms and the corresponding pore size distribution plots of various SnO2@graphene nanocomposites: (a and b) SG-120, (c and d) SG-140, (e and f) SG-160. | |
In order to better understand the surface chemical compositions of the SnO2@graphene nanocomposites, XPS spectra are further measured, as shown in Fig. 6. According to Fig. 6(a), only Sn, O and C elements are detected in the sample, indicating its high chemical purities. C1s of SG-140 is deconvolved into peaks related to different oxygen containing functional groups (Fig. 6(b)). The main peak located at about 284.5 eV is ascribed to non-oxygenated ring carbon atoms. While the other peaks at 286.2, 287.8 and 289.1 eV are assigned to the oxygen-containing groups (C–OH), (C
O) and (O
C–OH), respectively. These changes indicate that most of the oxygenated functional groups are successfully reduced and graphene is formed accordingly.43 O1s of SG-140 (Fig. 6(c)) is divided into two peaks located at 531.2 eV and 533.4 eV, which is due to the existence of the crystal lattice O2− and O(OH) (hydroxyl ions) species in the SnO2@graphene composites, respectively.44 Fig. 6(d) presents the separated XPS spectrum of Sn3d peaks. The binding energy of Sn3d3/2 and Sn3d5/2 are found at 487.9 eV and 495.9 eV, respectively, which belongs to the characteristics of SnO2 compounds.45–47
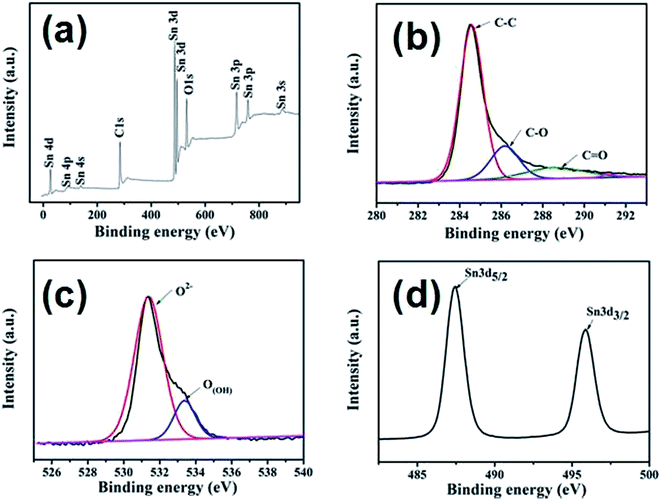 |
| Fig. 6 (a) survey scans spectra of SG-140, (b) separated spectra of C1s region, (c) separated spectra of O1s region, (d) spectra of Sn3d. | |
Fig. 7(a) shows the HRTEM image of SG-140 and the corresponding SAED pattern. The crystal lattice fringe of SnO2 with the interlayer distances of 0.334 nm is attributed to the spacing of the (110) planes of monoclinic SnO2 crystals in the nanocomposites.48,49 In order to further investigate the distribution and composition of the SG-140, the STEM image and the corresponding elemental mapping analysis are adopted. Fig. 7(b)–(d) show that Sn and O elements are homogeneously distributed throughout the samples, confirming the SnO2 nanocrystals are well distributed in the graphene matrix, in accordance with the TEM results.
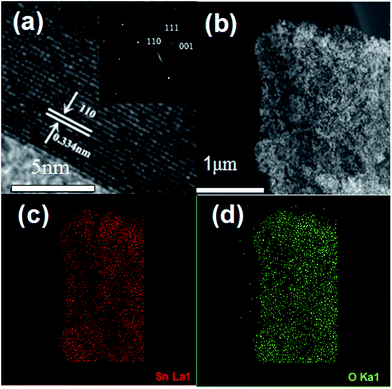 |
| Fig. 7 (a) HRTEM image (inset is the corresponding SAED pattern), (b) STEM image of SG-140, (c) and (d) the corresponding elemental mapping images. | |
TG curves of the three SnO2@graphene nanocomposites are displayed in Fig. 8. The weight loss at first is due to the absorbed water in the SnO2@graphene nanocomposites. All samples have a significant weight loss between 450 and 700 °C, because of the decomposition of graphene nanosheets. By calculation, the amount of SnO2 in the SG-120, SG-140, SG-160 nanocomposites is about 27.3%, 25.7%, 23.1%, respectively, as listed in Table 1.
 |
| Fig. 8 TG curves of various SnO2@graphene nanocomposites: (a) SG-120, (b) SG-140, (c) SG-160. | |
Table 1 The physicochemical properties of various SnO2@graphene nanocomposites synthesized at different reaction temperature
Sample |
SBETa (m2 g−1) |
Weight (%) |
Lengthb (nm) |
Charge (mA h g−1) |
Discharge (mA h g−1) |
The specific surface area calculated by the (Brunauer–Emmett–Teller) BET method. The size of SnO2@graphene estimated from TEM image. |
SG-120 |
610.5 |
27.3 |
18.7 |
980.4 |
1085.7 |
SG-140 |
544.2 |
25.7 |
28.4 |
1270.1 |
1284.9 |
SG-160 |
511.6 |
23.1 |
33.8 |
675.0 |
732.1 |
SnO2 |
6.6 |
|
20.3 |
5.2 |
5.2 |
2.6 Electrochemical performance
Fig. 9 displays the charge/discharge curves of (a) SG-120, (b) SG-140 and (c) SG-160 for the 1st, 10th, 50th and 100th cycles at a rate of 200 mA g−1, respectively. The discharge and charge capacity profiles of the same cell at various cycles are illustrated. With increasing cycles, the discharge capacity and the charge capacity of our materials decrease. The cell is first cycled at a current density of 200 mA g−1 for SG-140, a very high specific capacity of 1608 and 1024 mA h g−1 based on the total mass of the composites is seen, as depicted in Fig. 9(a) (red curves). These are larger than the theoretical capacity of SnO2 (782 mA h g−1) and our SnO2@graphene composites (Ctheo. = CSnO2 × %SnO2 + Cgraphene × %graphene = 782 × 25.7% + 774 × 74.3% = 776.1 mA h g−1; here, we regard the theoretical capacity of single layer graphene as 774 mA h g−1, which is related to the electrochemical reaction of Li2C6 = C6 + 2Li+ + 2e).50 Particularly, the capacity of the SnO2@graphene is more than the theoretical capacity even at a current density of 200 mA g−1 after 50 cycles. When the cycle increases to 100 cycles, our materials still could deliver a discharge capacity of 981 mA h g−1. Moreover, the first discharge and charge capacities of others are 732 and 675 mA h g−1 for SG-160, 1085 and 980 mA h g−1 for SG-120. It should be noteworthy that the discharge capacities of three SnO2@graphene nanocomposites are higher during the first cycle. After that, during the discharge process, SG-120, SG-140 and SG-160 samples still retain less capacities of the initial capacity, respectively. The capacity loss could be attributed to the irreversibility resulting from SnO2 reduction and the formation of solid electrolyte on the surface of the active material.
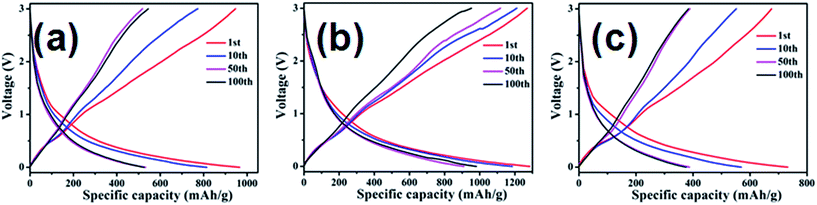 |
| Fig. 9 The charge–discharge curves of various SnO2@graphene nanocomposites: (a) SG-120, (b) SG-140, (c) SG-160 at a current density of 200 mA g−1. | |
As we known, the main reason for the rapidly fading of SnO2 electrode is the large volume expansion of the SnO2 material occurs during cycling, leading to the pulverization of the electrode.51,52 Fig. 10(a) shows the cycle performance of the three SnO2@graphene nanocomposites at a current density of 200 mA g−1. As expected, the SnO2@graphene nanocomposites exhibited a good cycling stability. Significantly, the SG-140 still exhibits a discharge capacity as high as 981 mA h g−1 after 100 cycles, higher than those of SG-120 (530 mA h g−1), SG-160 (377 mA h g−1), GO and pure SnO2. The superior cycling performance is mainly due to the rational growth of SnO2 nanoparticles onto the graphene matrix, which serves as a spacer that keeps the neighboring graphene separated. Moreover, the graphene could effectively prevent the volume expansion/contraction and aggregation of SnO2 nanoparticles during Li ions charge/discharge process.
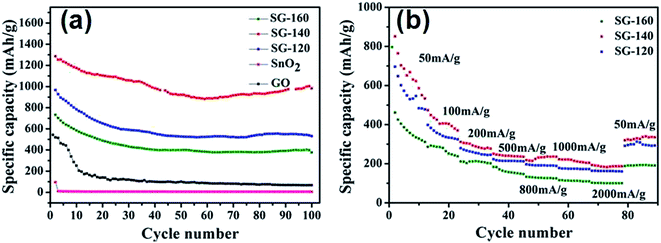 |
| Fig. 10 (a) Cycle performance of various SnO2@graphene nanocomposites at a current density of 200 mA g−1, (b) the rate capabilities of SnO2@graphene nanocomposites various current rates from 50 to 2000 mA g−1. | |
To investigate the rate capability, the SnO2@graphene nanocomposites are discharged and charged at various current rates from 50 to 2000 mA g−1. From the Fig. 9(b), the capacity of the SnO2@graphene nanocomposites decreases with improving the current density. Impressively, the rate capability of SG-140 is far better than that of others. Even at a high current density of 2000 mA g−1, the capacity of the SG-140 is still much higher than those of SG-120 and SG-160 up to approximately 186 mA h g−1. Furthermore, the reversible capacity can be recovered to a high value when the current density is returned to 50 mA g−1. The lithium storage properties of the hybrid electrodes are superior to the self-assembly of ordered, SnO2@graphene nanoporous composites prepared by reassembled process and in situ chemical methods. On one hand, the graphene with a good electrical conductivity can improve the electrical conductivity of the whole electrode, thus enhancing the rate capability.53,54 On the other hand, the relatively high specific BET surface area can increase the electrolyte–electrode interface and make the electrochemical reaction to easily carry out. In addition, the high reversible discharge capacity compared with the other SnO2 composites may also be due to the topological defects of our materials. As presented in Table 1, SG-140 material has a high ID/IG in Raman spectra. A large number of surface defects are introduced onto the graphene nanosheets during chemical exfoliation process, which leads to the formation of disordered carbon structure and further increases the active sites for lithium storage, further improving lithium intercalation properties. Besides, the rod-like SnO2 nanoparticles are homogeneously anchored onto the surface of graphene nanosheets, which could also provide parallel channels for Li+ ions intercalation through the surface with the least resistance to some extent.
Scheme 2 presents the illustration for bare SnO2 nanoparticle and the present SnO2@graphene composite electrodes during the insertion/extraction processes of lithium ions. The bare SnO2 nanoparticles undergo a large volumetric expansion during the lithiation process and cause pulverization of the electrode materials (Scheme 2a), thus resulting in capacity decay. While the volume expansion of the SnO2@graphene composites could be effectively restricted by the wrapped graphene substrate, Li+ ions insert into SnO2 nanoparticles (Scheme 2b). According to the BET data in Table 1, even though the volume expansion still happens, the graphene layers could facilitate the formation of 3D electronic conductive networks, provide a large number of active sites for Li-ion migration, enhance the conductivity and shorten the electronic transport route, and expand the gap between graphene layers, thus resulting in an easier migration for Li-ions. Finally, the short transport distance of lithium ions results in a reversible reaction of Li2O and Sn to yield SnO2. Consequently, the obtained SnO2@graphene nanocomposites demonstrate an excellent electrochemical property including a high lithium storage capability, good rate performance and long cycle life during the cycling.
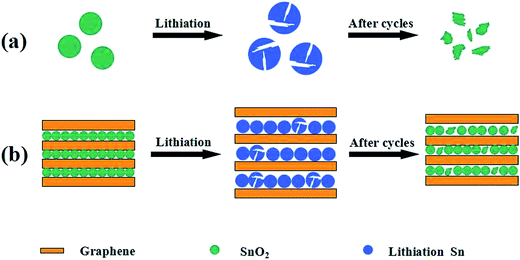 |
| Scheme 2 Diagram for the lithiation process of electrodes after many cycles: (a) bare SnO2, (b) SnO2@graphene nanocomposite. | |
3. Conclusions
In conclusion, we provide a facile hydrothermal method for fabrication of rod-like SnO2@graphene nanocomposites with large surface areas and well-defined morphology. The resulting rod-like SnO2 nanoparticles are homogeneously anchored onto the surface of graphene nanosheets. Additionally, the length of rod-like SnO2 nanoparticles can be readily adjusted by simply varying the reaction temperature. The electrochemical results indicate that the SnO2@graphene nanocomposites exhibit superior lithium ion battery performance with a large reversible capacity, excellent cyclic performance, and good rate capability. In particular, the obtained SnO2@graphene nanocomposites at 140 °C have a reversible discharge capacity as high as 981 mA h g−1 after 100 cycles at a current density of 200 mA g−1. The results indicate that the structure and morphology of SnO2 have a significant influence on its electrochemical performance. The present method can provide an alternative route for the design and fabrication of morphology-controlled other nanocrystals onto the graphene, which will extend their applications in the energy storage.
Acknowledgements
The work was financially supported by the National Natural Science Foundation of China (11275121, 21471096, 21371116), and Program for Innovative Research Team in University (IRT13078).
Notes and references
- P. Poizot, S. Laruelle, S. Grugeon, L. Dupont and J. M. Tarascon, Nano-sized transition-metal oxides as negative-electrode materials for lithium-ion batteries, Nature, 2000, 407, 496–499 CrossRef CAS PubMed
. - P. G. Bruce, B. Scrosati and J. M. Tarascon, Nanomaterials for rechargeable lithium batteries, Angew. Chem., Int. Ed., 2008, 47, 2930–2946 CrossRef CAS PubMed
. - B. Luo, B. Wang, M. Liang, J. Ning, X. Li and L. Zhi, Reduced graphene oxide-mediated growth of uniform tin-core/carbon-sheath coaxial nanocables with enhanced lithium ion storage properties, Adv. Mater., 2012, 24, 1405–1409 CrossRef CAS PubMed
. - B. Zhao, Y. Jiang, H. Zhang, H. Tao, M. Zhong and Z. Jiao, Morphology and electrical properties of carbon coated LiFePO4 cathode materials, J. Power Sources, 2009, 189, 462–466 CrossRef CAS
. - H. J. Zhang, H. H. Tao, Y. Jiang, Z. Jiao, M. H. Wu and B. Zhao, Ordered nanostructure CoO/CMK-3 nanocomposites as the anode materials for lithium-ion batteries, J. Power Sources, 2010, 195, 2950–2955 CrossRef CAS
. - W. Li, F. Wang, S. Feng, J. Wang, Z. Sun, B. Li, Y. Li, J. Yang, A. A. Elzatahry, Y. Xia and D. Zhao, Sol-gel design strategy for ultradispersed TiO2 nanoparticles on graphene for high-performance lithium ion batteries, J. Am. Chem. Soc., 2013, 135, 18300–18303 CrossRef CAS PubMed
. - K. Zhang, W. Zhao, J. T. Lee, G. Jang, X. Shi and J. H. Park, A magnetic field assisted self-assembly strategy towards strongly coupled Fe3O4 nanocrystal/rGO paper for high-performance lithium ion batteries, J. Mater. Chem. A, 2014, 2, 9636–9644 CAS
. - L. Tao, J. Zai, K. Wang, H. Zhang, M. Xu, J. Shen, Y. Su and X. Qian, Co3O4 nanorods/graphene nanosheets nanocomposites for lithium ion batteries with improved reversible capacity and cycle stability, J. Power Sources, 2012, 202, 230–235 CrossRef CAS
. - L. He, R. Ma, N. Du, J. Ren, T. Wong, Y. Li and S. T. Lee, Growth of TiO2 nanorod arrays on reduced graphene oxide with enhanced lithium-ion storage, J. Mater. Chem., 2012, 22, 19061–19066 RSC
. - S. Ding, J. S. Chen, D. Luan, F. Y. C. Boey, S. Madhavi and X. W. Lou, Graphene-supported anatase TiO2 nanosheets for fast lithium storage, Chem. Commun., 2011, 47, 5780–5782 RSC
. - N. Li, G. Zhou, R. Fang, F. Li and H. M. Cheng, TiO2/graphene sandwich paper as an anisotropic electrode for high rate lithium ion batteries, Nanoscale, 2013, 5, 7780–7784 RSC
. - F. Wei, H. Zhang, M. Nguyen, M. Ying, R. Gao and Z. Jiao, Template-free synthesis of flower-like SnO2 hierarchical nanostructures with improved gas sensing performance, Sens. Actuators, B, 2015, 215, 15–23 CrossRef CAS
. - J. Y. Huang, L. Zhong, C. M. Wang, J. P. Sullivan, W. Xu, L. Q. Zhang, S. X. Mao, N. S. Hudak, X. H. Liu, A. Subramanian, H. Fan, L. Qi, A. Kushima and J. Li, In situ observation of the electrochemical lithiation of a single SnO2 nanowire electrode, Science, 2010, 330, 1515–1520 CrossRef CAS PubMed
. - H. B. Wu, J. S. Chen, H. H. Hng and X. W. Lou, Nanostructured metal oxide-based materials as advanced anodes for lithium-ion batteries, Nanoscale, 2012, 4, 2526–2542 RSC
. - Y. Tang, D. Wu, S. Chen, F. Zhang, J. Jia and X. Feng, Highly reversible and ultra-fast lithium storage in mesoporous graphene-based TiO2/SnO2 hybrid nanosheets, Energy Environ. Sci., 2013, 6, 2447–2451 CAS
. - S. Yang, W. Yue, J. Zhu, Y. Ren and X. Yang, Graphene-based mesoporous SnO2 with enhanced electrochemical performance for lithium-ion batteries, Adv. Funct. Mater., 2013, 23, 3570–3576 CrossRef CAS
. - R. Chen, T. Zhao, T. Tian, S. Cao, P. R. Coxon, K. Xi, D. Fairen-Jimenez, R. V. Kumar and A. K. Cheetham, Graphene-wrapped sulfur/metal organic framework-derived microporous carbon composite for lithium sulfur batteries, APL Mater., 2014, 2, 124109 CrossRef
. - J. Hassoun, F. Bonaccorso, M. Agostini, M. Angelucci, M. G. Betti, R. Cingolani, M. Gemmi, C. Mariani, S. Panero and V. Pellegrini, An advanced lithium-ion battery based on a graphene anode and a lithium iron phosphate cathode, Nano Lett., 2014, 14, 4901–4906 CrossRef CAS PubMed
. - J. Sun, H.-W. Lee, M. Pasta, H. Yuan, G. Zheng, Y. Sun, Y. Li and Y. Cui, A phosphorene–graphene hybrid material as a high-capacity anode for sodium-ion batteries, Nat. Nanotechnol., 2015, 10, 980–985 CrossRef CAS PubMed
. - K. S. Novoselov, A. K. Geim, S. V. Morozov, D. Jiang, Y. Zhang, S. V. Dubonos and I. V. Grigorieva, Electric field effect in atomically thin carbon films, Science, 2004, 306, 666–669 CrossRef CAS PubMed
. - E. Quesnel, F. Roux, F. Emieux, P. Faucherand, E. Kymakis, G. Volonakis, F. Giustino, B. Martín-García, I. Moreels and S. A. Gürsel, Graphene-based technologies for energy applications, challenges and perspectives, 2D Mater, 2015, 2, 030204 CrossRef
. - M. D. Stoller, S. Park, Y. Zhu, J. An and R. S. Ruoff, Graphene based ultracapacitors, Nano Lett., 2008, 8, 3498–3502 CrossRef CAS PubMed
. - K. I. Bolotin, K. J. Sikes, Z. Jiang, M. Klima, G. Fudenberg, J. Hone, P. Kim and H. L. Stormer, Ultrahigh electron mobility in suspended graphene, Solid State Commun., 2008, 146, 351–355 CrossRef CAS
. - C. Lee, X. Wei, J. W. Kysar and J. Hone, Measurement of the elastic properties and intrinsic strength of monolayer graphene, Science, 2008, 321, 385–388 CrossRef CAS PubMed
. - L. Jaber-Ansari, K. P. Puntambekar, H. Tavassol, H. Yildirim, A. Kinaci, R. Kumar, S. J. Saldaña, A. A. Gewirth, J. P. Greeley, M. K. Y. Chan and M. C. Hersam, Defect evolution in graphene upon electrochemical lithiation, ACS Appl. Mater. Interfaces, 2014, 6, 17626–17636 CAS
. - H. Zhang, P. Xu, Y. Ni, H. Geng, G. Zheng, B. Dong and Z. Jiao, In situ chemical synthesis of SnO2/reduced graphene oxide nanocomposites as anode materials for lithium-ion batteries, J. Mater. Res., 2014, 29, 617–624 CrossRef CAS
. - S. Ding, D. Luan, F. Y. C. Boey, J. S. Chen and X. W. Lou, SnO2 nanosheets grown on graphene sheets with enhanced lithium storage properties, Chem. Commun., 2011, 47, 7155–7157 RSC
. - H. Liu, J. Huang, X. Li, J. Liu, Y. Zhang and K. Du, Flower-like SnO2/graphene composite for high-capacity lithium storage, Appl. Surf. Sci., 2012, 258, 4917–4921 CrossRef CAS
. - G. Ji, B. Ding, Z. Sha, J. Wu, Y. Ma and J. Y. Lee, Conformal graphene encapsulation of tin oxide nanoparticle aggregates for improved performance in reversible Li+ storage, Nanoscale, 2013, 5, 5965–5972 RSC
. - S. Li, W. Xie, S. Wang, X. Jiang, S. Peng and D. He, Facile synthesis of rGO/SnO2 composite anodes for lithium ion batteries, J. Mater. Chem. A, 2014, 2, 17139–17145 CAS
. - Y. Xu, H. Bai, G. Lu, C. Li and G. Shi, Flexible graphene films via the filtration of water-soluble noncovalent functionalized graphene sheets, J. Am. Chem. Soc., 2008, 130, 5856–5857 CrossRef CAS PubMed
. - H. Zhang, P. Xu, G. Du, Z. Chen, K. Oh, D. Pan and Z. Jiao, A facile one-step synthesis of TiO2/graphene composites for photodegradation of methyl orange, Nano Res., 2011, 4, 274–283 CrossRef CAS
. - H. Zhang, Q. He, X. Zhu, D. Pan, X. Deng and Z. Jiao, Surfactant-free solution phase synthesis of monodispersed SnO2 hierarchical nanostructures and gas sensing properties, CrystEngComm, 2012, 14, 3169–3176 RSC
. - X. Han, M. Jin, S. Xie, Q. Kuang, Z. Jiang, Y. Jiang, Z. Xie and L. Zheng, Synthesis of tin dioxide octahedral nanoparticles with exposed high-energy (221) facets and enhanced gas-sensing properties, Angew. Chem., Int. Ed., 2009, 121, 9344–9347 CrossRef
. - Y. N. Ko, S. B. Park and Y. C. Kang, Design and fabrication of new nanostructured SnO2-carbon composite microspheres for fast and stable lithium storage performance, Small, 2014, 10, 3240–3245 CrossRef CAS PubMed
. - X. Liu, J. Cheng, W. Li, X. Zhong, Z. Yang, L. Gu and Y. Yu, Superior lithium storage in a 3D macroporous graphene framework/SnO2 nanocomposite, Nanoscale, 2014, 6, 7817–7822 RSC
. - P. G. Ren, D. X. Yan, X. Ji, T. Chen and Z. M. Li, Temperature dependence of graphene oxide reduced by hydrazine hydrate, Nanotechnology, 2011, 22, 055705 CrossRef PubMed
. - Y. Li, X. Lv, J. Lu and J. Li, Preparation of SnO2-nanocrystal/graphene-nanosheets composites and their lithium storage ability, J. Phys. Chem. C, 2010, 114, 21770–21774 CAS
. - Y. Huang, D. Wu, J. Wang, S. Han, L. Lv, F. Zhang and X. Feng, Amphiphilic polymer promoted assembly of macroporous graphene/SnO2 frameworks with tunable porosity for high-performance lithium storage, Small, 2014, 10, 2226–2232 CrossRef CAS PubMed
. - X. Jiang, X. Yang, Y. Zhu, H. Jiang, Y. Yao, P. Zhao and C. Li, 3D nitrogen-doped graphene foams embedded with ultrafine TiO2 nanoparticles for high-performance lithium-ion batteries, J. Mater. Chem. A, 2014, 2, 11124–11133 CAS
. - X. Dong, L. Li, C. Zhao, H. K. Liu and Z. Guo, Controllable synthesis of RGO/FexOy nanocomposites as high-performance anode materials for lithium ion batteries, J. Mater. Chem. A, 2014, 2, 9844–9850 CAS
. - F. H. Du, Y. S. Liu, J. Long, Q. C. Zhu, K. X. Wang, X. Wei and J. S. Chen, Incorporation of heterostructured Sn/SnO nanoparticles in crumpled nitrogen-doped graphene nanosheets for application as anodes in lithium-ion batteries, Chem. Commun., 2014, 50, 9961–9964 RSC
. - H. Xu, S. Yuan, Z. Wang, Y. Zhao, J. Fang and L. Shi, Graphene anchored with ZrO2 nanoparticles as anodes of lithium ion batteries with enhanced electrochemical performance, RSC Adv., 2014, 4, 8472–8480 RSC
. - J. Zhang, Z. Xiong and X. S. Zhao, Graphene–metal-oxide composites for the degradation of dyes under visible light irradiation, J. Mater. Chem., 2011, 21, 3634–3640 RSC
. - L. S. Zhang, L. Y. Jiang, H. J. Yan, W. D. Wang, W. Wang, W. G. Song, Y. G. Guo and L. J. Wan, Mono dispersed SnO2 nanoparticles on both sides of single layer graphene sheets as anode materials in Li-ion batteries, J. Mater. Chem., 2010, 20, 5462–5467 RSC
. - H. Zhang, M. Ying, R. Gao, L. Hu, Z. Jiao and X. Zhu, Carbon-mediated fabrication of core–shell structured SnO2@TiO2 nanocomposites with excellent photocatalytic performance, RSC Adv., 2015, 5, 58439–58448 RSC
. - H. Xu, L. Shi, Z. Wang, J. Liu, J. Zhu, Y. Zhao, M. Zhang and S. Yuan, Fluorine-doped tin oxide nanocrystal/reduced graphene oxide composites as lithium ion battery anode material with high capacity and cycling stability, ACS Appl. Mater. Interfaces, 2015, 7, 27486–27493 CAS
. - S. Ding, D. Luan, F. Y. C. Boey, J. S. Chen and X. W. Lou, SnO2 nanosheets grown on graphene sheets with enhanced lithium storage properties, Chem. Commun., 2011, 47, 7155–7157 RSC
. - X. Zhou, L. J. Wan and Y. G. Guo, Binding SnO2 nanocrystals in nitrogen-doped graphene sheets as anode materials for lithium-ion batteries, Adv. Mater., 2013, 25, 2152–2157 CrossRef CAS PubMed
. - G. Wang, B. Wang, X. Wang, J. Park, S. Dou, H. Ahn and K. Kim, Sn/graphene nanocomposite with 3D architecture for enhanced reversible lithium storage in lithium ion batteries, J. Mater. Chem., 2009, 19, 8378–8384 RSC
. - L. Li, A. Kovalchuk and J. Tour, SnO2-reduced graphene oxide nanoribbons as anodes for lithium ion batteries with enhanced cycling stability, Nano Res., 2014, 7, 1319–1326 CrossRef CAS
. - W. Sun and Y. Wang, Graphene-based nanocomposite anodes for lithium-ion batteries, Nanoscale, 2014, 6, 11528–11552 RSC
. - M. Armand and J. M. Tarascon, Building better batteries, Nature, 2008, 451, 652–657 CrossRef CAS PubMed
. - D. Wang, J. Yang, X. Li, D. Geng, R. Li, M. Cai, T. K. Sham and X. Sun, Layer by layer assembly of sandwiched graphene/SnO2 nanorod/carbon nanostructures with ultrahigh lithium ion storage properties, Energy Environ. Sci., 2013, 6, 2900–2906 CAS
.
|
This journal is © The Royal Society of Chemistry 2016 |
Click here to see how this site uses Cookies. View our privacy policy here.