DOI:
10.1039/C6RA01591C
(Paper)
RSC Adv., 2016,
6, 31661-31667
Improved photocatalytic activity of RGO/MoS2 nanosheets decorated on TiO2 nanoparticles†
Received
19th January 2016
, Accepted 16th March 2016
First published on 17th March 2016
Abstract
In this work we have synthesized a heterogeneous hybrid junction of reduced graphene oxide (RGO)/MoS2 and hybrid composites of TiO2–RGO/MoS2 using a hydrothermal method. The hybrid composites were characterized using UV-visible spectroscopy, scanning electron microscopy (SEM), X-ray photoelectron spectroscopy (XPS), Raman spectroscopy, and electron paramagnetic resonance spectroscopy (EPR). Raman spectroscopy displays that the hybrid junction was formed between two dimensional RGO and few layer MoS2 nanosheets. In the TiO2–RGO/MoS2 hybrid composite, EPR studies show that the lattice Ti3+ signal was decreased and at the same time equivalent amounts of the O˙− signal increased under UV light irradiation. These results suggested that the interfacial charge transferred from the TiO2 surface to the MoS2 surface active sites directly or through the RGO nanosheets toward suppressing the recombination rate of the electron–hole pairs for an enhanced methylene blue degradation. RGO behaves like a conductor, it accepted only electrons and transported to the active sites through separating the potential level distance between the two semiconductors.
1. Introduction
Nowadays, clean energy is in demand due to its low cost and abundant source. Solar hydrogen production is one of the best ways to get clean energy from available semiconductors by the reduction of water splitting.1 Titanium dioxide (TiO2) is the most explored and applied photocatalyst.1,2 It has a strong oxidizing power, high stability, low-cost, an abundant source and is relatively non-toxic. TiO2 exists in mainly three phases as anatase, rutile and brookite. The anatase phase has been shown to be the 100% metastable state with a band gap near 3.2 eV.3 Under UV light irradiation charge carriers are generated in the valence band (VB) edge and conduction band (CB) edge of TiO2 towards the oxidation and reduction reactions.4 However, the electron–hole recombination rate is faster and mainly responsible for its limited application in catalysis. Therefore, it is necessary to overcome this problem by charge separation through suppressing the recombination rate of the electron–hole pairs.5
A series of strategies have been developed to synthesize TiO2 based nanocomposites by doping metals (Ag,6 Au,5 Pt7) or non-metals (B,8 N,8,9 P,10 F,11 S,12 C13) into the TiO2 lattice to inhibit the recombination rate and narrow the band gap. It has been reported that TiO2 nanoparticles coupled with narrow band gap semiconductors (CuO,14 MoS2,15 WO3,16 and Fe2O3 (ref. 17)) can improve the interfacial charge transfer (IFCT) and inhibit the recombination rate of charge carriers. In addition to this, TiO2 heterostructures have gained much interest with two-dimensional materials for photocatalytic as well as electrocatalytic applications. Chang et al.18 reported that TiO2 nanoparticles coupled with inorganic heterostructures of graphene and molybdenum disulfide (MoS2) nanosheets were an effective way to increase the oxidation and reduction reactions.
Single layer two dimensional graphene nanosheets have the excellent ability of electron acceptance and transport to the semiconductor–graphene junction.19,20 However, chemically exfoliated graphene oxide (GO) exhibits poor conduction and electrical properties. Pei et al.21 reported that reduced graphene oxide (RGO) shows better properties than GO through removing the surface attached oxygen-containing functional groups to restore the sp2-hybridized network. In particular, molybdenum disulfide (MoS2) with a layered structure has been extensively used in electrocatalysis, fuel cells, lubricants, and optoelectronic devices as well as in lithium storage batteries.18,22–26 Thurston et al.23 reported that bulk MoS2 was turned into monolayer or few layers, at the same time its band gap also changed from an indirect to direct band gap. Monolayer or few layer MoS2 nanosheets exhibited an improved number of active sites and conductivity. Recently, Xie’s group demonstrated that a novel defect-rich structure introduced the additional active sites into the MoS2 ultrathin nanosheets which significantly improved the electrocatalytic performance.27,28 Some contradicting models were proposed for photogenerated charge transfer from the TiO2 to the MoS2 surface and vice versa under light irradiation to account for the enhanced catalytic activity.15,23,25,29 No unified direct spectroscopic evidence has been reached to suggest the charge carriers direction, which pointed to the relative position of the CB and VB edges in the two semiconductors.
Herein, we report anatase phase TiO2 nanoparticles coupled with few layers of MoS2 nanosheets (TiO2–MoS2) and a RGO/MoS2 hybrid junction (TiO2–RGO/MoS2) using hydrothermal processes under basic conditions. The EPR spectra illustrated that the interfacial charge transferred from the TiO2 nanoparticles to the MoS2 surface via the RGO nanosheets. RGO behaves like a conductor; it accepts only electrons and transports them to the active sites of the MoS2 cocatalyst surface under UV light irradiation. The TiO2–RGO/MoS2 composite showed an enhanced charge carrier separation in order to improve the photocatalytic activity towards the degradation of methylene blue dye. Overall, the EPR studies provided us further understanding of the charge transportation and recombination pathways mechanism to design and synthesise graphene-based materials for energy applications.
2. Experimental
2.1 Materials
Anatase phase TiO2 nanoparticles (Hombikat UV100) were obtained from World Chem. Industry, Taipei, Taiwan. Graphite powder (99.9995%), Na2MoO4·2H2O and thiourea were purchased from Alfa Aesar. NaNO3, H2O2 (30–31%), NaBH4 and N2H4 (64–65%) were purchased from Sigma Aldrich. H2SO4 from Fluka and KMnO4 obtained from J. T. Baker were used as oxidizing agents. Double distilled water was used in all the experiments. All the materials were used as received.
2.2 Characterizations
Scanning electron microscopy (SEM) images were obtained from a JEOL JSM7000F field emission scanning electron microscope. X-ray photoelectron spectroscopy (XPS) characterizations were performed using a Kα system (Thermo Scientific) with monochromatic Al Kα excitation and a charge neutralizer. Hybrid junction investigations were performed using Raman Spectroscopy (Renishaw). X-Band Electron Paramagnetic Resonance (EPR) spectra were recorded at 77 K using a Bruker EMX spectrometer with a TE102 cavity. The EPR sample tube was immersed in a liquid nitrogen-containing finger Dewar and a Newport 1000 W xenon lamp with an IR filter was used as an irradiation light source to transmit into the EPR cavity through an optical fiber of the same time rate irradiation. The UV-visible diffuse reflectance and photocatalytic activity were recorded on a Shimadzu (UV-2550) UV-vis spectrophotometer.
2.3 Experimental methods
2.3.1 Synthesis of the RGO/MoS2 hybrid junction.
A two-dimensional layered MoS2 catalyst was synthesized according to the previous literature.27 In brief, 1 mmol of Na2MoO4·2H2O and 7.5 mmol of thiourea were dissolved in 60 ml of distilled water. The homogeneous solution was stirred for a few minutes and transferred into a 100 ml Teflon-lined autoclave at 210 °C for 24 h. A black precipitate was collected using centrifugation, washed with deionized water and ethanol, and dried at 80 °C overnight in a vacuum oven. In order to prepare the RGO/MoS2 hybrid junction, 4 mg of the prepared MoS2 catalyst was added to a water and ethanol mixture and exfoliated for 1 h using sonication. The obtained large size particles were removed through low speed centrifugation to obtain supernatant as few layers of MoS2 nanosheets. To the above supernatant 1 mg of RGO was added and kept for 1 h under sonication and stirred for 5 h accordingly to make a homogeneous solution. The solution was transferred to an autoclave at 180 °C for 10 h and gradually cooled down to room temperature. The obtained suspension was used as the hybrid junction of few layers RGO/MoS2 nanosheets.
2.3.2 Synthesis of the TiO2–RGO/MoS2 hybrid nanocomposite.
All the hybrid nanocomposites were prepared using a hydrothermal method. In a typical procedure, 400 mg of anatase phase TiO2 nanoparticles was dispersed in the above water and ethanol mixture containing the hybrid junction of RGO/MoS2 nanosheets. The mixture was sonicated for a few minutes and stirred for 5 h at room temperature to prepare a homogeneous solution. After that the solution was transferred to an autoclave for hydrothermal treatment at 180 °C for 10 h. The autoclave was naturally cooled down to room temperature and washed repeatedly with distilled water and ethanol, then dried at 80 °C. Other samples were also synthesized using the same procedure without RGO and varying the (0, 1) wt% concentration of the MoS2 nanosheets.
2.4 Photocatalytic degradation
The hybrid composites photocatalytic activities were evaluated using the degradation of aqueous solutions of methylene blue (MB) under natural sunlight irradiation. The catalyst (50 mg) was added into 100 ml of MB dye solution (10 mg l−1 aqueous solution) under ambient conditions. The solution was stirred for 20 min in the dark to get the adsorption–desorption equilibrium between the catalyst and dye solution. There was not much difference observed due to the graphene or MoS2 nanosheets. The solution was exposed to sunlight (10 am to 12 pm) under constant stirring. At a given 20 min time interval, 3 ml aliquots of the solution were withdrawn and centrifuged to remove the catalyst. The concentration of MB in the aqueous solution was determined with the help of a UV-vis spectrophotometer. All sample activity was done with the same time under sunlight irradiation. The experiments were carried out at the National Dong Hwa University, Hualien, Taiwan (23°53’38.06”N, 121°32’36.88”E). The surrounding temperature was 25 ± 2 °C while the solution temperature increased to 28 ± 5 °C during the irradiation time.
3. Results and discussion
3.1 UV-visible spectroscopy
UV-visible spectroscopy is employed to estimate the band gap of the as prepared semiconductors. Fig. 1(a) shows two band peaks at 627 nm and 672 nm that are attributed to the direct excitonic transitions of A1 and B1 in the dispersion of the exfoliated MoS2 nanosheet and the RGO/MoS2 hybrid junctions. These observed characteristic peaks suggest that the energy splitting in the MoS2 valence band is due to spin–orbit coupling.26,30 It has been stated that as MoS2 turns from bulk to monolayer or few layers, its band gap also changes from an indirect to direct band gap transition.23 However, monolayer MoS2 exhibited an improved number of active sites and conductivity.27 Another characteristic band appears at 230–300 nm, corresponding to a π–π* plasmon peak of the reduced graphene oxide. The diffuse reflectance spectra of TiO2/MoS2 and the TiO2–RGO/MoS2 hybrid composite are shown in Fig. 1(b). The optical absorbance increased with the increase in MoS2 concentration, whereas the band gap is slightly narrowed in the RGO/MoS2 hybrid junction. This could be attributed to chemical bonding between TiO2–RGO due to the formation of Ti–O–C bonds.
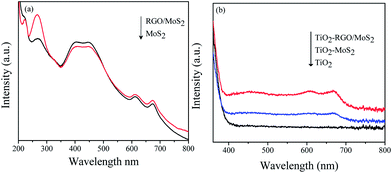 |
| Fig. 1 UV-visible spectra of the as-prepared (a) dispersion of the RGO/MoS2 hybrid junction and (b) the TiO2–RGO/MoS2 heterogeneous hybrid composites. | |
3.2 Morphology analysis
The morphology and microscopic structure of the as-prepared MoS2 catalyst are characterized using SEM as shown in Fig. 2(a). The as prepared MoS2 catalyst shows a nanoflower like structure obtained with an average diameter size of 1–2 μm. The surface of these nanoflowers possesses massive petals, which are free and closely aggregated. It could be seen that the petals grown on the surface of the MoS2 flowers are disorderly intersected together and point toward a common center of the sphere to form the spherical structure.18 These nanoflowers are separated using sonication and used as a few layers MoS2 catalyst for the formation of the TiO2–RGO/MoS2 composite as shown in Fig. 2(b). In the hydrothermal method, these few layered MoS2 and RGO nanosheets are easily decorated on TiO2 nanoparticles which can be seen in the TEM and HR-TEM images (ESI Fig. 2(a) and (b)†).
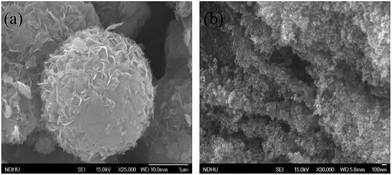 |
| Fig. 2 SEM images of (a) the MoS2 nanoflower and (b) TiO2–RGO/MoS2 hybrid composite. | |
3.3 XPS spectroscopy
High resolution X-ray photoelectron spectroscopy (XPS) is performed to study the oxidation state of the prepared samples such as the RGO, MoS2 and TiO2–RGO/MoS2 composites. Chemically exfoliated graphene oxide shows binding energies at 284.4 eV and 286.6 eV which correspond to the C–C and C–O bonds, respectively21,31 as shown in Fig. 3(a). However, graphene oxide exhibited poor conduction and electrical properties due to the oxygen-containing functional groups present on the surface. Fig. 3(b) shows the binding energy at 284.4 eV denoted as C 1s which suggested that reduced graphene oxide is formed by a reduction process.21 The as prepared MoS2 nanoflower shows two peaks at 228.9 and 232.1 eV assigned to the Mo 3d5/2 and Mo 3d3/2, respectively and the valence state was determined as Mo4+.28 Moreover, the S 2p peaks at 161.5 and 162.7 eV are equivalent to the S2− valence state of MoS2 (ESI Fig. 4(a) and (b)†).
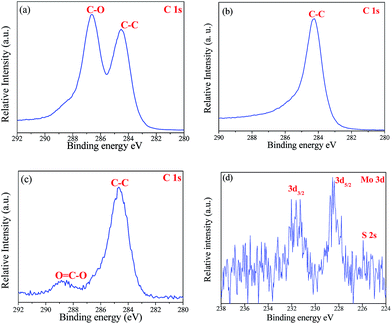 |
| Fig. 3 XPS spectra of the as prepared (a) graphene oxide (b) reduced graphene oxide; and in the TiO2–RGO/MoS2 hybrid composite (c) C 1s of RGO and (d) Mo 3d. | |
In the hydrothermal method, TiO2 nanoparticles are decorated with a RGO/MoS2 nanosheet junction in order to form the TiO2RGO/MoS2 hybrid composites. The high surface area of RGO supported the Ti–O–C bond formation between the TiO2 nanoparticles and graphene nanosheets.15 RGO exhibited two peaks at 284.4 eV and 289 eV which are ascribed to the C–C and O
C–O bonds as shown in Fig. 3(c). The O
C–O bonds appeared, mainly due to the formation of Ti–O–C bonds as well as the partially oxidized RGO nanosheets with the TiO2 nanoparticles.15 On the other side, the small amounts of observed binding energy at 228.8 eV and 231.4 eV imply typical values for Mo4+ in MoS2 as shown in Fig. 3(d). There is no evidence found of Mo6+ valence states in MoO3, which proved the molybdenum atom does not partially oxidize in the TiO2–RGO/MoS2 hybrid composite.25
3.4 Raman spectroscopy
Raman spectroscopy is a useful technique to analyse the vibrational mode and the formation of the RGO and RGO/MoS2 nanosheets hybrid junction. Fig. 4(a) shows the strong Raman features of the D band at 1343 cm−1 and the G band at 1596 cm−1 originate with additional lower intensity bands at 2500 cm−1 and 2700 cm−1 related to RGO.15,24,32,33 In the RGO/MoS2 hybrid junction, two characteristic peaks are ascribed to the MoS2 site at 381 cm−1 of the lower energy E12g (in plane vibration of two ‘S’ atom with respect to the Mo atom) and 407 cm−1 of the higher energy A1g (out of plane vibration of S atom) Raman modes.22,26 Simultaneously lower intensity peaks of the D and G bands are displayed showing the existence of reduced graphene oxide with chemical bonding as shown in Fig. 4(b). Li et al.22 reported that the graphene/MoS2 nanosheet junction exhibited excellent HER catalytic activity. It has been reported that Raman mode spacing between E12g and A1g showed the layer dependence of MoS2 nanosheets. It is worth noting that two peaks in the monolayer or few layers are coming towards each other while in the bulk they are shifting away from each other. The observed shifting difference between the two peaks ∼26 cm−1 shows the few layers of MoS2, which is consistent with the reported literature.26,34–36
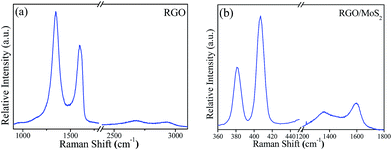 |
| Fig. 4 Raman spectra of the as prepared (a) RGO and (b) RGO/MoS2 heterogeneous hybrid junction. | |
3.5 EPR spectroscopy
3.5.1 Photoinduced electron transfer.
Electron paramagnetic resonance spectroscopy (EPR) is a pivotal tool for investigating the paramagnetic defect species, and study their dynamics.
To investigate the interfacial charge transfer mechanism, we compared all the recorded EPR spectra of (bare) TiO2, TiO2–MoS2 and the TiO2–RGO/MoS2 composite at 77 K under UV-light irradiation as shown in Fig. 5. In the TiO2 nanoparticles, under light irradiation, electrons are excited from the valence band (VB) edge to the conduction band (CB) edge leaving positively charged holes. The EPR spectra of the TiO2 nanoparticles exhibited two major signals in the VB edge and CB edge due to the trapped holes and electrons respectively. Above the Fermi level these electrons are free and a few of them trapped in defect sites leading to originate the paramagnetic cations Ti3+ signal while the remaining electrons continued staying on the surface of the nanoparticles. The sharp and intense Ti3+ signal B at g⊥ = 1.991 and g∥ = 1.961 clearly suggested that electrons trapped in the lattice defect sites lie a few electron volts below the CB edge. On the other hand, the observed O˙− signal A at g values (2.025, 2.016, and 2.003) are attributed to the photogenerated holes trapped on the surface or subsurface bridging oxygen atom sites of the TiO2 nanoparticles. The observed values are in good agreement with previous literature values, which independently found for O˙− and Ti3+ signals in anatase phase TiO2.4,9,10,37,38 Reduced graphene oxide does not show any paramagnetic impurity signal except the free electron signal at g = 2.003 (data not added here). These signals could have appeared due to the existence of a defect rich surface in the RGO nanosheets.39 It could be possible that dangling bonds are created on the surface of the RGO, such types of bonds usually occur on the surface of carbon–carbon bonding.39,40 However, the observed signal does not change which clearly suggesting that the RGO behaves like a conductor under light irradiation.
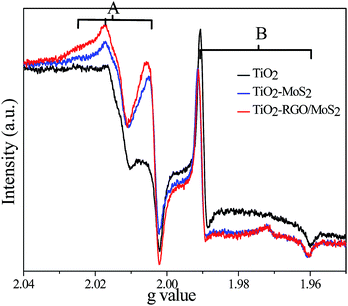 |
| Fig. 5 EPR spectra were recorded after 5 min. UV light irradiation at 77 K TiO2 (black color), TiO2–MoS2 (blue color) and TiO2–RGO/MoS2 (red color). | |
The EPR spectra clearly displayed that bare (uncoupled) TiO2 (black-color) nanoparticles are created a large amount of charge carriers (electrons and holes) in their appropriate bands as shown in signals A and B. In the TiO2–MoS2 composites, the obtained signal intensity of B has noticeably decreased, and at the same time signal A intensity is increased (5-blue color). This implies that the few layered MoS2 redox potential is slightly lower than the TiO2 conduction band. Thermodynamically, the photogenerated electrons quickly migrated to the lower potential of the MoS2 surface in order to trap in the TiO2 defect sites. Later, these transferred electrons are stored on the MoS2 surface in which a few of them could trap on defect sites. On the other hand holes are accumulated at the VB edge of TiO2 by charge carriers separation. In this hybrid composite, the MoS2 surface has low electron mobility, less electrical conductivity as well as less surface area which could affect the smaller amount of conduction electrons transferred to the MoS2 active sites.
In the TiO2–RGO/MoS2 composite, the signal intensity of B is decreased as compared to the bare TiO2 nanoparticles, where simultaneously the signal intensity of A is remarkably increased as shown in Fig. 5-(red color). It suggests that a high concentration of photoinduced electrons are transferred from the TiO2 surface to the MoS2 surface in order to improve charge carriers separation. On the RGO nanosheets, these transported electrons have very high mobility and shuttling ability, they quickly migrated on the MoS2 surface active sites. However, a Mo5+ (nearly g = 1.93) signal could not be observed and ensure that molybdenum atoms do not partially oxidised36 which is in accordance with the XPS data Fig. 3(d). There could be two pathways that the photogenerated electrons can be transferred from the TiO2 surface to the few layer MoS2 surface either directly or through the RGO nanosheets. Min et al.41 reported that RGO and MoS2 greatly enhanced the electronic conductivity of the nanohybrid which is beneficial to the photoinduced electron transfer during the illumination. RGO also supports the formation of the maximum percentage of junction between the TiO2 nanoparticles and MoS2 nanosheets. In these heterogeneous junctions, the electron transfer driving force is mainly dependent on their energy difference and the coupling distance between the donor–acceptor semiconductors.
3.5.2 Charge carrier separation.
In addition to this, we studied the emission of the decay process or the charge recombination pathways of all the prepared hybrid composites as shown in Fig. 6. After UV light irradiation was turned off, the charge transfer process suddenly stopped and the available CB photoinduced electrons quickly followed the recombination process with the VB holes through reduction of signal A and B. In the TiO2–RGO/MoS2 hybrid composite, large amounts of O˙− signal A is detected which clearly suggests that the holes are still localized in the VB edge of the TiO2 nanoparticles, whereas the photoinduced electrons and holes followed a much slower recombination rate than that of the other samples. It is obvious that once electrons transferred from the TiO2 CB to the RGO or MoS2 nanosheets surface, then thermodynamically it has very less probability to recombine with the VB holes in TiO2. Here, the graphene sheet behaves like a conductor which played a crucial role for separating the potential level distance between the two semiconductors.
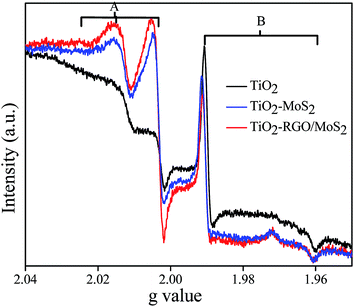 |
| Fig. 6 EPR spectra were recorded after 10 min. UV light irradiation turned off at 77 K TiO2 (black color), TiO2–MoS2 (blue color) and TiO2–RGO/MoS2 (red color). | |
From Fig. 5 and 6, TiO2–MoS2 and the TiO2–RGO/MoS2 hybrid composites displayed that the lattice trapped electrons Ti3+ signal B and the localized holes O˙− signal A are consistently fluctuated upon the light irradiation being turned on or off. It warrants that “light screening or shading effect” does not occurred with a low concentration of the RGO and MoS2 cocatalyst.14 However, the TiO2 nanoparticles are covered by a high loading concentration of layered MoS2 or the RGO/MoS2 cocatalyst; in which they could absorb light and reduce the photo-excitation capacity of TiO2 to occurred the “light screening effect or shading effect” in a smaller fraction.
3.6 Photocatalytic performance
The photocatalytic activity of all the prepared samples was evaluated using methylene blue (MB) degradation as shown in Fig. 7. Under sunlight irradiation all samples band gap are activated through the generation of electron–hole pairs for redox reactions. It is clear that the TiO2–RGO/MoS2 composite exhibits an excellent photocatalytic activity better than the bare TiO2 nanoparticles and TiO2–MoS2 composite. We believe that the observed catalytic activity comes from the interfacial charge transferred to the MoS2 surface active sites and simultaneous holes accumulating at the VB edge of TiO2. These transferred electrons on the MoS2 active sites and the accumulated holes in the TiO2 valence band edge are mainly participating in degradation of MB molecules. In this composite RGO supports to keep away the potential level distance between two semiconductors of TiO2 and MoS2 towards inhibiting the recombination rate of the electron–hole pairs.
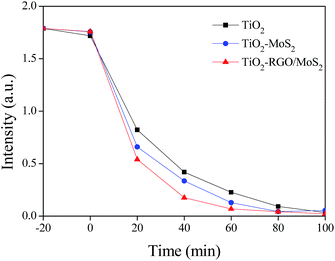 |
| Fig. 7 Photocatalytic degradation of methylene blue on various catalysts under sunlight irradiation. | |
A plausible mechanism for the charge transfer in the solid–solid interface of TiO2–MoS2 and the TiO2–RGO/MoS2 hybrid composite is proposed in Scheme 1. Under UV light irradiation, both the TiO2 and MoS2 bands are activated through the generation of electrons and holes in their appropriate bands (eqn (1)). EPR data supports that the photoinduced electrons (decrease in trapped electrons Ti3+ signal) are transferred from the TiO2 nanoparticles surface to the few layered MoS2 nanosheets surface via either a direct route or through the RGO junction. On the other hand the increased O˙− signal confirms that the holes are accumulated in the TiO2 valence band edge by enhanced charge carrier separation (eqn (2)). In this hybrid composite, the RGO sheet separated the potential level distance between two semiconductors towards suppressing the recombination rate of electron–hole pairs. The injected electrons are stored or captured in the MoS2 surface active sites. These separated charge carriers could migrate to the surface of the photocatalysts, where they rapidly react with H2O, O2 and hydroxide ions to generate ˙OH radicals and superoxide radical anions O2˙− as indicated in eqn (3) and (4). Finally, the created powerful oxidizing agents H2O2 and the hydroxyl radicals ˙OH could effectively decompose the methylene blue molecules.29 Holes and radicals can decomposing pollutants via the released CO2 (eqn (8)). The related chemical reactions can be expressed as:
| TiO2–RGO/MoS2 + hν → TiO2 (e− + h+)–RGO/MoS2 (e− + h+) | (1) |
| TiO2 (h+)–RGO (e−)/MoS2 (e− + h+) → TiO2 (h+)–RGO/MoS2 (e−) (e− + h+) | (2) |
| O2− + H2O → ˙HO2 + OH− | (5) |
| ˙HO2 + H2O → H2O2 + ˙OH | (6) |
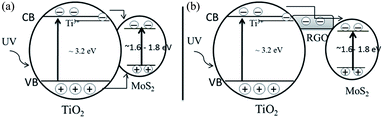 |
| Scheme 1 Schematic diagram shows the charge carriers transfer mechanism under UV light irradiation (a) TiO2–MoS2 and (b) TiO2–RGO/MoS2 hybrid composite. | |
4. Conclusions
In summary, we have successfully synthesized a RGO/MoS2 heterogeneous junction and a TiO2–RGO/MoS2 composite using a hydrothermal method. The characterization techniques demonstrated that the heterogeneous hybrid junctions were formed between the RGO and the few layers of MoS2 nanosheets. In the TiO2–RGO/MoS2 hybrid composite, EPR studies illustrated that the photoinduced electrons (decreased Ti3+ signal) were transferred from the TiO2 nanoparticles to the few layered MoS2 nanosheets surface either directly or through the RGO interface. At the same time the holes (increased O˙− signal) were accumulated in the TiO2 valence band edge, suggesting that a charge carrier separation process occurred. In this composite, RGO played key roles; it accepted only electrons and transported them to the MoS2 surface catalytically active edge sites. It is believed that the enhanced photo activity of the MB degradation could come from the improved interfacial charge transfer, increased number of catalytically active edge sites, and the slower recombination rate of the electron–hole pairs.
Conflict of interest
The authors declare no competing financial interests.
Acknowledgements
The National Science Council of Taiwan (Grant NSC-104-2627-M-259-001) supported this research.
References
- M. Gratzel, Nature, 2001, 414, 338–344 CrossRef CAS PubMed.
- A. Fujishima and K. Honda, Nature, 1972, 238, 37–38 CrossRef CAS PubMed.
- H. G. Yang, C. H. Sun, S. Z. Qiao, J. Zou, G. Liu, S. C. Smith, H. M. Cheng and G. Q. Lu, Nature, 2008, 453, 638–641 CrossRef CAS PubMed.
- C. P. Kumar, N. O. Gopal, T. C. Wang, M.-S. Wong and S. C. Ke, J. Phys. Chem. B, 2006, 110, 5223–5229 CrossRef CAS PubMed.
- Z. Bian, T. Tachikawa, P. Zhang, M. Fujitsuka and T. Majima, J. Am. Chem. Soc., 2014, 136, 458–465 CrossRef CAS PubMed.
- T. Hirakawa and P. V. Kamat, J. Am. Chem. Soc., 2005, 127, 3928–3934 CrossRef CAS PubMed.
- B. K. Vijayan, N. M. Dimitrijevic, J. Wu and K. A. Gray, J. Phys. Chem. C, 2010, 114, 21262–21269 CAS.
- N. O. Gopal, H.-H. Lo and S.-C. Ke, J. Am. Chem. Soc., 2008, 130, 2760–2761 CrossRef CAS PubMed.
- H.-H. Lo, N. O. Gopal, S.-C. Sheu and S.-C. Ke, J. Phys. Chem. C, 2014, 118, 2877–2884 CAS.
- N. O. Gopal, H.-H. Lo, T.-F. Ke, C.-H. Lee, C.-C. Chou, J.-D. Wu, S.-C. Sheu and S.-C. Ke, J. Phys. Chem. C, 2012, 116, 16191–16197 CAS.
- A. M. Czoska, S. Livraghi, M. Chiesa, E. Giamello, S. Agnoli, G. Granozzi, E. Finazzi, C. D. Valentin and G. Pacchioni, J. Phys. Chem. C, 2008, 112, 8951–8956 CAS.
- P. V. R. K. Ramacharyulu, D. B. Nimbalkar, J. P. Kumar, G. K. Prasad and S.-C. Ke, RSC Adv., 2015, 5, 37096–37101 RSC.
- G. Wu, T. Nishikawa, B. Ohtani and A. Chen, Chem. Mater., 2007, 19, 4530–4537 CrossRef CAS.
- G. Li, N. M. Dimitrijevic, L. Chen, T. Rajh and K. A. Gray, J. Phys. Chem. C, 2008, 112, 19040–19044 CAS.
- Q. Xiang, J. Yu and M. Jaroniec, J. Am. Chem. Soc., 2012, 134, 6575–6578 CrossRef CAS PubMed.
- H. Tada, A. Kokubu, M. Iwasaki and S. Ito, Langmuir, 2004, 20, 4665–4670 CrossRef CAS PubMed.
- M. R. Dhananjeyan, E. Mielczarski, K. R. Thampi, P. Buffat, M. Bensimon, A. Kulik, J. Mielczarski and J. Kiwi, J. Phys. Chem. B, 2001, 105, 12046–12055 CrossRef CAS.
- K. Chang, Z. Mei, T. Wang, Q. Kang, S. Ouyang and J. Ye, ACS Nano, 2014, 8, 7078–7087 CrossRef CAS PubMed.
- A. K. Geim and K. S. Novoselov, Nat. Mater., 2007, 6, 183–191 CrossRef CAS PubMed.
- I. V. Lightcap, T. H. Kosel and P. V. Kamat, Nano Lett., 2010, 10, 577–583 CrossRef CAS PubMed.
- S. Pei and H.-M. Cheng, Carbon, 2012, 50, 3210–3228 CrossRef CAS.
- Y. Li, H. Wang, L. Xie, Y. Liang, G. Hong and H. Dai, J. Am. Chem. Soc., 2011, 133, 7296–7299 CrossRef CAS PubMed.
- T. R. Thurston and J. P. Wilcoxon, J. Phys. Chem. B, 1999, 103, 11–17 CrossRef CAS.
- K. Zhang, H.-J. Kim, X. Shi, J.-T. Lee, J.-M. Choi, M.-S. Song and J. H. Park, Inorg. Chem., 2013, 52, 9807–9812 CrossRef CAS PubMed.
- W. Zhou, Z. Yin, Y. Du, X. Huang, Z. Zeng, Z. Fan, H. Liu, J. Wang and H. Zhang, Small, 2013, 9, 140–147 CrossRef CAS PubMed.
- Y. Yao, L. Tolentino, Z. Yang, X. Song, W. Zhang, Y. Chen and C.-P. Wong, Adv. Funct. Mater., 2013, 23, 3577–3583 CrossRef CAS.
- J. Xie, H. Zhang, S. Li, R. Wang, X. Sun, M. Zhou, J. Zhou, X. W. Lou and Y. Xie, Adv. Mater., 2013, 25, 5807–5813 CrossRef CAS PubMed.
- J. Xie, J. Zhang, S. Li, F. Grote, X. Zhang, H. Zhang, R. Wang, Y. Lei, B. Pan and Y. Xie, J. Am. Chem. Soc., 2013, 135, 17881–17888 CrossRef CAS PubMed.
- W. Ho, J. C. Yu, J. Lin, J. Yu and P. Li, Langmuir, 2004, 20, 5865–5869 CrossRef CAS PubMed.
- L. A. King, W. Zhao, M. Chhowalla, D. J. Riley and G. Eda, J. Mater. Chem. A, 2013, 1, 8935–8941 CAS.
- C.-J. Liu, S.-Y. Tai, S.-W. Chou, Y.-C. Yu, K.-D. Chang, S. Wang, F. S.-S. Chien, J.-Y. Lin and T.-W. Lin, J. Mater. Chem., 2012, 22, 21057–21064 RSC.
- J. Shen, B. Yan, M. Shi, H. Ma, N. Li and M. Ye, J. Mater. Chem., 2011, 21, 3415–3421 RSC.
- X. Yu, J. Zhang, Z. Zhao, W. Guo, J. Qiu, X. Mou, A. Li, J. P. Claverie and H. Liu, Nano Energy, 2015, 16, 207–217 CrossRef CAS.
- M. A. Lukowski, A. S. Daniel, F. Meng, A. Forticaux, L. Li and S. Jin, J. Am. Chem. Soc., 2013, 135, 10274–10277 CrossRef CAS PubMed.
- Y. Liu, H. Nan, X. Wu, W. Pan, W. Wang, J. Bai, W. Zhao, L. Sun, X. Wang and Z. Ni, ACS Nano, 2013, 7, 4202–4209 CrossRef CAS PubMed.
- L. Cai, J. He, Q. Liu, T. Yao, L. Chen, W. Yan, F. Hu, Y. Jiang, Y. Zhao, T. Hu, Z. Sun and S. Wei, J. Am. Chem. Soc., 2015, 137, 2622–2627 CrossRef CAS PubMed.
- T. Berger, M. Sterrer, O. Diwald, E. Knözinger, D. Panayotov, T. L. Thompson and J. T. Yates, J. Phys. Chem. B, 2005, 109, 6061–6068 CrossRef CAS PubMed.
- Y. Nakaoka and Y. Nosaka, J. Photochem. Photobiol., A, 1997, 110, 299–305 CrossRef CAS.
- C. V. Pham, M. Krueger, M. Eck, S. Weber and E. Erdem, Appl. Phys. Lett., 2014, 104, 132102 CrossRef.
- S. Cui, H. Pu, E. C. Mattson, Z. Wen, J. Chang, Y. Hou, C. J. Hirschmugl and J. Chen, Anal. Chem., 2014, 86, 7516–7522 CrossRef CAS PubMed.
- S. Min and G. Lu, J. Phys. Chem. C, 2012, 116, 25415–25424 CAS.
Footnote |
† Electronic supplementary information (ESI) available: Synthesis, XRD patterns, FE-SEM images, XPS spectra and photocatalytic degradation of MB absorbance spectra. See DOI: 10.1039/c6ra01591c |
|
This journal is © The Royal Society of Chemistry 2016 |
Click here to see how this site uses Cookies. View our privacy policy here.