DOI:
10.1039/C6RA01709F
(Paper)
RSC Adv., 2016,
6, 31161-31166
Luminescent sensing from a new Zn(II) metal–organic framework†
Received
20th January 2016
, Accepted 10th March 2016
First published on 14th March 2016
Abstract
A new metal–organic framework having the formula (NH2(CH3)2)[Zn2(OAc)(L)]·0.5DMF (H4L = 2,5-di(3′,5′-dicarboxylphenyl)pyridine) (GDMU-3) has been synthesized and characterized. The net of GDMU-3 is uninodal and is closely related to the lvt net, which has the same Schläfli symbol of 42·84. GDMU-3 displays selective properties for the detection of nitrobenzene and Fe3+ ions. Remarkably, GDMU-3 exhibits an excellent capability to adsorb methylene blue with high selectivity. The present work indicates that GDMU-3 could be a potential candidate for developing novel luminescence sensors for the selective sensing of nitrobenzene which can be deployed for explosives, Fe3+ and organic dyes.
Introduction
The luminescent properties of metal–organic frameworks (MOFs) have attracted considerable attention for some time; naturally, these properties of luminescent MOFs (LMOFs) can potentially be used for real-world applications.1–3 Recently, chemical sensors for fast and highly selective detection of high explosives and heavy metal ions have attracted increasing attention concerning environmental and humanitarian implications.4–8 The advantages and challenges of LMOF-based luminescent sensors are well summarized by several previous review articles.9,10 In principle, the spectroscopic characteristics of LMOFs can potentially be used as a sensing signal.11 Out of the several spectroscopic characteristics, the change in intensity of fluorescent emission is the most commonly observed change.12 It has been also speculated that the electronic nature of a molecule may affect the electron transfer or energy transfer between guest molecules and LMOFs.13–15 Metal ions, such as Mn2+, Fe3+, and Cu2+, are also capable of quenching fluorescence since they can induce ligand–metal charge transfer and relax the excitation energy through a non-radiative pathway.16–19 However, it is a significant and challenging task to synthesize new materials for fluorescence detection of toxic solvents and heavy metal ions.20
The selection of ligand plays a crucial role in design and construction of stable LMOFs for fluorescence detection.21 We selected 2,5-di(3′,5′-dicarboxylphenyl)pyridine (H4L) and Zn(II) to synthesize MOFs for detection of explosives and heavy metal ions. The choice of the ligand and Zn(II) stems from the following reasons.22–24 (1) Numerous explosives are comprised of good electron acceptors having electron-deficient –NO2 groups, which are the common chemical constituents of commercial explosives. (2) H4L is a rigid dicarboxylate ligand with aromatic rings and nitrogen atoms with lone-pair electrons, so these N atoms as functional sites are predicted to recognize metal ions. (3) In addition, d10 metal ions usually show high complexation affinity to carboxylate and do not interfere with fluorescence, because they can display varied coordination numbers and geometries, and exhibit outstanding luminescent properties.24
With these considerations, herein we report a new MOF, namely (NH2(CH3)2)[Zn2(OAc)(L)]·0.5DMF (GDMU-3), that exhibits a 3D net with Schläfli symbol of 42·84. Investigations of the luminescence property of GDMU-3 emulsions in different solvents and its selective sensing of Fe3+ ions and methylene blue (MB) are also reported. Also, efforts have been made to address the quenching mechanism for nitrobenzene detection.
Materials and methods
All chemicals were purchased from Jinan Henghua Sci. & Tec. Co. Ltd without further purification. Powder X-ray diffraction (PXRD) was conducted with a Bruker D8 ADVANCE X-ray diffractometer with Cu-Kα radiation (λ = 1.5418 Å) at 50 kV, 20 mA with a scanning rate of 6° min−1 and a step size of 0.02°. Simulated powder patterns were calculated using Mercury 2.0. The purity and homogeneity of the bulk products were determined by comparison of the simulated and experimental PXRD patterns. Fourier transform infrared (IR) spectra were measured using a Nicolet Impact 750 FTIR spectrometer in the range of 400–4000 cm−1 and with KBr pellet samples. Thermogravimetric analysis was performed under air atmosphere from room temperature to 800 °C at a heating rate of 10 °C min−1, using an SDT Q600 thermogravimetric analyzer. Energy dispersive X-ray spectroscopy (EDS) data were obtained with a Philips XL-30 scanning electron microscope.
X-ray crystallography
Room-temperature single-crystal X-ray diffraction data collection was carried out using a Bruker SMART APEX diffractometer that was equipped with graphite monochromated Mokα radiation (λ = 0.71073 Å) by using an ω-scan technique. The intensities were corrected for absorption effects by using SADABS. The structures were solved by using SHELXL2014.25 Absorption corrections were applied by using non-hydrogen atoms were refined anisotropically. For GDMU-3, the unit cell exhibits large regions that are occupied by solvent molecules; the solvent molecules could not be modeled. The SQUEEZE option in PLATON26 was used to produce a set of solvent-free diffraction intensities. The nature and number of solvent molecules were established from CHN elemental analyses and thermogravimetric analyses. Crystallographic details and selected bond dimensions for GDMU-3 are listed in Tables 1 and 2. CCDC number: 1437346.
Table 1 Crystal data and structure refinement information for compound GDMU-3
R = ∑(Fo − Fc)/∑(Fo). wR2 = {∑[w(Fo2 − Fc2)2]/∑(Fo2)2}1/2. |
Formula weight |
1033 |
Crystal system |
Monoclinic |
Space group |
C2/c |
Crystal color |
Colorless |
a, Å |
33.109(2) |
b, Å |
7.9520(5) |
c, Å |
18.4662(12) |
β, ° |
111.0306(10) |
V, Å3 |
4538.0(5) |
Z |
4 |
ρcalcd, g cm−3 |
1.416 |
μ, mm−1 |
0.991 |
F(000) |
1380 |
θ range, deg |
2.25–25.98 |
Reflections collected |
11 624 |
Independent reflections (Rint) |
0.0555 |
Reflections with I > 2σ(I) |
4432 |
R1, wR2 (I > 2σ(I))a |
0.0348, 0.0934 |
R1, wR2 (all data)b |
0.0451, 0.0981 |
Table 2 Selected bond distances (Å) and angles (deg) of structure of GDMU-3
Zn1–O3 |
1.938(2) |
Zn1–O2 |
1.961(2) |
Zn1–O5 |
1.963(2) |
Zn1–O1 |
1.988(2) |
O3–Zn1–O2 |
115.17(8) |
O3–Zn1–O5 |
112.50(9) |
O2–Zn1–O5 |
112.19(9) |
O3–Zn1–O1 |
95.04(7) |
O2–Zn1–O1 |
111.43(8) |
O5–Zn1–O1 |
109.20(9) |
Synthesis of (NH2(CH3)2)[Zn2(OAc)(L)]·0.5DMF (GDMU-3)
A mixture of Zn(OAc)2·2H2O (0.220 g), H4L (0.018 g) and DMF (4 mL) was placed in a screw-capped vial. Then two drops of HNO3 (63%, aq.) were added into the mixture. The vial was capped and placed in an oven at 105 °C for 3 days. The resulting colorless single crystals obtained were washed with absolute CH3CH2OH three times to get GDMU-3. Anal. calcd for C26.5H24.5N2.5O10.5Zn2 (%): C, 47.03; H, 3.65; N, 5.17. Found (%): C, 47.22; H, 3.52; N, 5.20. IR (KBr, cm−1): 3402 (vs); 2918 (m); 1621 (vs); 1560 (v); 1415 (v); 1226 (m); 1042 (m); 720 (v); 530 (m). 13C NMR (126 MHz, DMSO-d6) δ 166.33 (–COOH), 166.28 (–COO−), 153.12 (–C7), 147.23 (–C11), 138.14 (–C13), 137.32 (–C4,3,2), 136.98 (–C14,18), 133.79 (–C9), 132.34 (–C10), 132.09 (–C14,18), 131.60 (–C1,5), 131.35 (–C15,17), 130.68 (–C16), 129.69 (–C6), 121.42 (–C8), 40.02 (DMSO), 39.95 (–CH3–N), 39.85 (DMSO), 39.78 (–CH2–COO−), 39.69 (DMSO), 39.52 (DMSO), 39.35 (DMSO), 39.19 (DMSO), 39.02 (DMSO).
Results and discussion
The structure of GDMU-3 contains Zn2 units in which the two metal atoms are connected by carboxylates from two L ligands and a bridging acetate ligand (Fig. 1a). The tetrahedral geometry of each Zn(II) is then completed by L carboxylate in a monodentate manner, indicating that each dinuclear complex is coordinated by four different L ligands. Each L is, in turn, coordinated to four clusters, meaning that both the clusters and the ligands act as 4-connecting nodes. Such connectivity modes give rise to a 3D structure packed along the ab-plane (Fig. 1b). The overall 4-connected net is shown in Fig. 1c. The net is uninodal and is closely related to the lvt net,27 which has the same Schläfli symbol of 42·84. Both nets contain channels of eclipsed 4-membered rings which share edges with helical channels. The lvt net is non-centric and channels on opposite sides of the 4-membered rings have the same handedness (those on adjoining sides have the opposite handedness). The net defined by GDMU-3 is centrosymmetric and thus those on opposite sides of the 4-membered ring are of opposite handedness, while each side adjoins one of the same handedness and one of the opposite handedness. There exist two types of pores having dimensions 5.8 × 3.8 Å along the b axis and 10.2 × 20.5 Å along the c axis, respectively. These pores are filled with the terminal acetate, dimethylammonium and DMF molecules (Fig. S1†). As a consequence, the solvent-accessible voids, as calculated by using PLATON26 for GDMU-3 without the contribution of disordered DMF and dimethylammonium, amount to a volume of 2026.3 Å3 and represent 44.7% of the total volume of the structure. More importantly, the dimethylammonium anions are derived from the decomposition of DMF molecules and were used to balance the full charge of GDMU-3. This was also confirmed by the 13C NMR results, as shown in Fig. S2.†
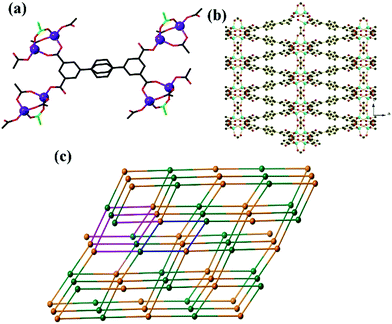 |
| Fig. 1 (a) Local coordination geometries of the ligands and Zn2 clusters in the structure of GDMU-3. The central ring of the L ligand is disordered over two positions, and the acetate ligand of the cluster is highlighted in green (all other carboxylates belong to L ligands). (b) View of the 3D net along the ab-plane. (c) The underlying 42·84 net in the structure of GDMU-3. Green nodes represent the 4-connecting ligands, while orange nodes represent the 4-connected Zn2 clusters. A 4-membered ring is highlighted in blue, while an adjoining helical channel is highlighted in pink. | |
Thermogravimetric analysis
The thermogravimetric analysis of GDMU-3 indicated two weight loss steps (Fig. S3†). The first weight loss begins at 25 °C and is completed at 127 °C. The observed weight loss of 4.6% corresponds to the loss of free DMF molecules (calcd 5.3%). The second weight loss can be attributed to the elimination of [NH2(CH3)2]+ cations and organic ligands. The final residual mass of 25.8% is roughly consistent with the formation of ZnO (calcd 24.1%).
Photoluminescence measurements
Since GDMU-3 is composed of the luminescent rigid ligand and the ZnII center having configuration d10,28 it shows strong luminescence with emission peak at 428 nm (λex = 320 nm). This emission band can be assigned to H4L ligand-centered emission, because the emission for the free H4L was observed at 375 nm (λex = 312 nm) (Fig. S4†). The enhancement in the emission intensity of GDMU-3 may arise from the aggregation-induced emission, where the coordination of the ligand and the metal ions can reduce the freedom of the ligands and their non-radiative transitions.29,30 This interaction shifts the energy levels for H4L, as confirmed by the UV-vis-NIR absorption spectrum for GDMU-3 (Fig. S5†).
In addition, the fluorescence properties of GDMU-3 in different solvent emulsions were investigated (Fig. 2). It has been reported that photoluminescence intensities are largely dependent on the solvent molecules, particularly in the case of nitrobenzene (NB),31–35 which exhibits significant quenching behavior. The physical interaction of the solute and solvent plays a pivotal role in such fluorescence behavior. To explore the sensing sensitivity of GDMU-3 towards NB in more detail, suspensions of GDMU-3 with gradually increasing concentration of NB in DMF were prepared to monitor the emissive response (Fig. 3). A finely ground powder of GDMU-3 was dispersed in the solution (Fig. S3†). This enables NB to be closely adhered to the surface of the MOF particles and facilitates possible host–guest interactions; as a result electron transfer from the electron-donating framework to the highly electron-deficient NB molecule can take place upon excitation, resulting in fluorescence quenching.36,37 The luminescence intensity decreased to 50% at 75 ppm, and complete quenching was observed at 175 ppm. The emission in NB exhibited a quenching effect. The observed quenching may be due to an inner filter effect as NB can absorb the excitation light. On the other hand, the –NO2 group attached to the aromatic ring makes it electron deficient in nature.38–40 Amongst the different solvents used in the investigation, NB shows a strong absorbing range from 260 to 410 nm, while other solvents have no significant absorption band in this range. As shown in Fig. S11,† the strong absorption band of L is located at ∼260 nm, which is largely overlapped by the electronic absorption band of NB. Upon excitation, there may be a competition for the absorption of the light source energy between NB and L. Combined with the electronic absorption and luminescence spectra, it may be suggested that the energy absorbed by L is transferred to the NB molecules, thereby resulting in the decrease in luminescence intensity, even quenching, of GDMU-3. The quenching mechanism is in agreement with that proposed in previous reports.32,40
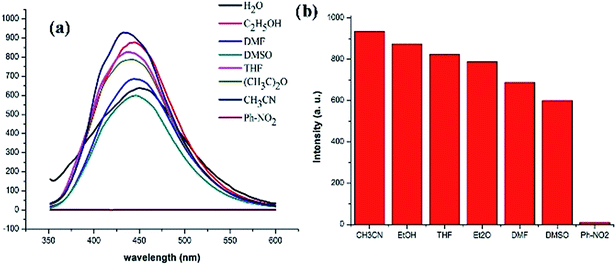 |
| Fig. 2 (a) Emission spectra of GDMU-3 in different solvents. (b) Emission intensity in different solvents (λex = 320 nm). | |
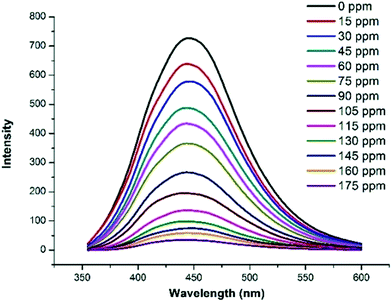 |
| Fig. 3 Emission spectra of GDMU-3 at different nitrobenzene concentrations (λex = 320 nm). | |
The luminescence spectra of GDMU-3 dispersed in DMF solutions (3 mL) of metal ions were also studied (Fig. 4). Fig. 4a indicates that metal ions with saturated electron configurations do not lead to a significant change in the luminescent intensity; in contrast, the other metal cations, especially Fe3+,41 can bring about essentially complete quenching of the emission intensity. These results demonstrate that compound GDMU-3 can be a highly effective and selective luminescent sensor for Fe3+ ions. As illustrated in Fig. 4c, the luminescence intensity of GDMU-3 is almost completely quenched at an Fe(NO3)3 concentration of 10−2 M. According to the Stern–Volmer equation, I0/I = 1 + Ksv[M],42 the quenching coefficient Ksv of 4926 M−1 is obtained from the luminescent data. The decreases in intensity are caused by Fe3+ ion-induced ligand-to-metal charge transfer and provides a non-radiative pathway for the excitation energy to transfer to the ground state. As reported by Zheng,43 Fe3+ shows strong absorption from 320 nm to 400 nm, which exhibit a wide overlap with the emission spectra of GDMU-3, indicating the existence of energy transfer. As confirmed by the PXRD patterns (see Fig. S6†), the crystal structures of GDMU-3 immersed in metal ion solutions remain unchanged. The framework integrity of the metal-incorporated GDMU-3 samples was confirmed by PXRD patterns (Fig. S6†) and EDS analysis of GDMU-3–Fe3+ indicated that the sample has an Fe
:
Zn ratio of 0.5 (Fig. S7†). We speculate that the recognition of Fe3+ ions might be related to the interaction between the Fe3+ ions and the N atoms of pyridyl rings in GDMU-3. These observations are similar to those for previously reported MOFs.19,23 To elucidate the possible mechanism for such luminescence quenching by metal cations, N 1s X-ray photoelectron spectroscopy studies were carried out on GDMU-3 and GDMU-3@Fe3+. The N 1s peak from free pyridyl nitrogen atoms at 401.6 eV in GDMU-3 is shifted to 402.4 eV on the addition of Fe3+ (Fig. S8†), indicating the weak binding of pyridyl nitrogen atoms to Fe3+ in GDMU-3@Fe3+.
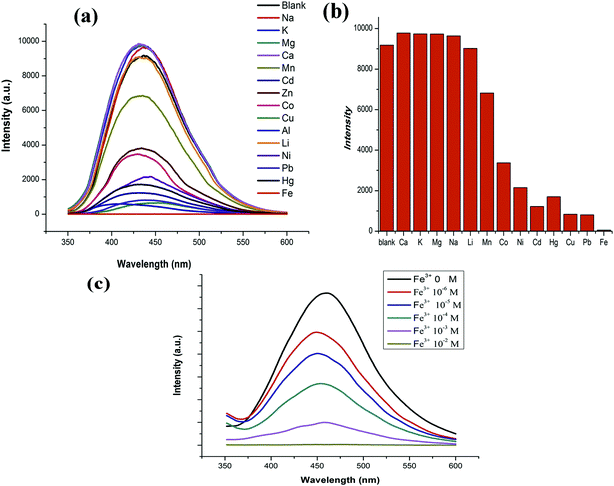 |
| Fig. 4 (a) Emission spectra of GDMU-3 with different metal ions. (b) Emission intensity with different metal ions. (c) Emission spectra of GDMU-3 with different Fe3+ ion concentrations (λex = 320 nm). | |
The removal of dyes from effluents before discharge into natural bodies is extremely important from an environmental point of view.44 To investigate whether compound GDMU-3 has the ability to adsorb dye molecules, we used it to capture dyes from DMF solutions. Dye molecules with different charges (see Fig. S9†) were selected. The samples of GDMU-3 were soaked in DMF solutions containing two dyes: MB and solvent yellow 2. It was observed that the MB dye molecules could be efficiently adsorbed over a period of time (2 h) and the colorless crystals of GDMU-3 gradually became colored, while the solvent yellow 2 could not be incorporated efficiently (Fig. 5). The ability of GDMU-3 to adsorb dyes from DMF solution was evaluated through UV/vis spectroscopy. Spectroscopic investigations of the supernatants showed that GDMU-3 can effectively incorporate cationic dyes into its networks, whereas neutral dyes were left in the supernatants. The absorptivity of GDMU-3 is 0.35 mg g−1 for MB and is 0.08 mg g−1 for solvent yellow 2. The selectivity of GDMU-3 for dyes could be attributed to the anionic framework, in which the [(CH3)2NH2]+ cations can be exchanged with cationic dyes. Preliminary investigations suggest that GDMU-3 may have potential application for removal of dyes from effluents.44–46 To confirm that selective absorption is due to ionic interaction of dye with the anionic framework, a dye releasing experiment was performed in DMF and a saturated solution of NaCl in DMF measured by UV/vis spectroscopy. This showed that, under the action of NaCl, the MB dye molecules in GDMU-3 can be gradually released (see Fig. S10†), while in DMF without NaCl the dye molecules are hardly released. Hence, we can safely conclude that selective absorption is due to ionic interaction of dyes with the anionic framework.47–50
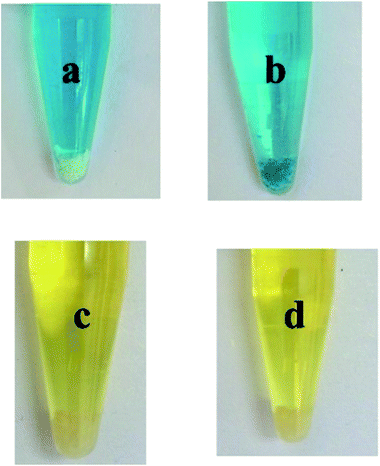 |
| Fig. 5 Dye-selective adsorption of GDMU-3. Photographs of dye solutions before (a and c) and after (b and d) interacting with materials. | |
Summary and conclusions
In summary, a new net of GDMU-3 is uninodal and is closely related to the lvt net. The compound is a potential candidate for developing novel luminescence sensors for the selective sensing of nitrobenzene which can be deployed for explosives, Fe3+ and organic dyes. These remarkable preliminary results provide us with an impetus to develop new metal–organic framework materials which find important and multifarious application for selective adsorption of cations and dyes and their separation.
Acknowledgements
This work was partially supported by grants from the National Natural Science Foundation of China (21201044), the Chinese Training Plan of Guangdong Province Outstanding Young Professors in Higher Education Institutions (grant YQ2013084), Innovation Training Project of Guangdong Medical University (2014ZYDM001, XJ105711445, 2014ZYDM018 and 2014ZZDM001), and College Students Innovation Training Project of Guangdong Province (201410571060, 201510571054, 2015010571048, 201510571076, 201410571053). Thanks are due to Dr S. B Batten. AK is grateful to DST, New Delhi for financial support in the form of project no. SB/FT/CS-018/2012.
References
- S. R. Zhang, D. Y. Du, J. S. Qin, S. J. Bao, S. L. Li, W. W. He, Y. Q. Lan, P. Shen and Z. M. Su, Chem.–Eur. J., 2014, 20, 3589 CrossRef CAS PubMed.
- Z. C. Hu, B. J. Deibert and J. Li, Chem. Soc. Rev., 2014, 43, 5815 RSC.
- D. Zacher, O. Shekhah, C. Woll and R. A. Fischer, Chem. Soc. Rev., 2009, 38, 1418 RSC.
- O. Shekhah, J. Liu, R. A. Fischer and C. Woll, Chem. Soc. Rev., 2011, 40, 1081 RSC.
- Y. Q. Xiao, Y. Cui, Q. Zheng, S. C. Xiang, G. D. Qian and B. L. Chen, Chem. Commun., 2010, 46, 5503 RSC.
- B. Chen, L. B. Wang, Y. Q. Xiao, F. R. Fronczek, M. Xue, Y. J. Cui and G. D. Qian, Angew. Chem., Int. Ed., 2009, 48, 500 CrossRef CAS PubMed.
- S. W. Thomas, G. D. Joly and T. M. Swager, Chem. Rev., 2007, 107, 1339 CrossRef CAS PubMed.
- A. Lan, K. Li, H. Wu, D. H. Olson, T. J. Emge, W. Ki, M. Hong and J. Li, Angew. Chem., Int. Ed., 2009, 48, 2334 CrossRef CAS PubMed.
- Y. Cui, Y. Yue, G. Qian and B. Chen, Chem. Rev., 2012, 112, 1126 CrossRef CAS PubMed.
- L. E. Kreno, K. Leong, O. K. Farha, M. Allendorf, R. P. Van Duyne and J. T. Hupp, Chem. Rev., 2012, 112, 1105 CrossRef CAS PubMed.
- J. He, M. Zha, J. Cui, M. Zeller, A. D. Hunter, S.-M. Yiu, S.-T. Lee and Z. Xu, J. Am. Chem. Soc., 2013, 135, 7807–7810 CrossRef CAS PubMed.
- Q. Tang, S. Liu, Y. Liu, J. Miao, S. Li, L. Zhang, Z. Shi and Z. Zheng, Inorg. Chem., 2013, 52, 2799 CrossRef CAS PubMed.
- S. Yoon, E. W. Miller, Q. He, P. H. Do and C. J. Chang, Angew. Chem., Int. Ed., 2007, 46, 6658 CrossRef CAS PubMed.
- G. G. Shan, H. B. Li, H. Z. Sun, D. X. Zhu, H. T. Cao and Z. M. Su, J. Mater. Chem. C, 2013, 1, 1440 RSC.
- P. Wu, J. Wang, C. He, X. Zhang, Y. Wang, T. Liu and C. Duan, Adv. Funct. Mater., 2012, 22, 1698 CrossRef CAS.
- S. R. Zhang, D. Y. Du, K. Tan, J. S. Qin, H. Q. Dong, S. L. Li, W. W. He, Y. Q. Lan, P. Shen and Z. M. Su, Chem.–Eur. J., 2013, 19, 11279 CrossRef CAS PubMed.
- K. Jayaramulu, R. P. Narayanan, S. J. George and T. K. Maji, Inorg. Chem., 2012, 51, 10089 CrossRef CAS PubMed.
- J. X. Ma, X. F. Huang, X. Q. Song and W. S. Liu, Chem.–Eur. J., 2013, 19, 3590 CrossRef CAS PubMed.
- J. C. Jin, L. Y. Pang, G. P. Yang, L. Hou and Y. Y. Wang, Dalton Trans., 2015, 44, 17222 RSC.
- Y. Salinas, R. Martinez-Manez, M. D. Marcos, F. Sancenon, A. M. Castero, M. Parra and S. Gil, Chem. Soc. Rev., 2012, 41, 1261 RSC.
- J. X. Ma, X. F. Huang, X. Q. Song and W. S. Liu, Chem.–Eur. J., 2013, 19, 3590 CrossRef CAS PubMed.
- S. Pramanik, C. Zheng, X. Zhang, T. J. Emge and J. Li, J. Am. Chem. Soc., 2011, 133, 4153 CrossRef CAS PubMed.
- B. Liu, W. P. Wu, L. Hou and Y. Y. Wang, Chem. Commun., 2014, 50, 8731 RSC.
- F. Y. Yi, W. T. Yang and Z. M. Sun, J. Mater. Chem., 2012, 22, 23201 RSC.
- G. M. Sheldrick, Acta Crystallogr., Sect. A: Cryst. Phys., Diffr., Theor. Gen. Crystallogr., 2008, 64, 112 CrossRef CAS PubMed.
- A. L. Spek, J. Appl. Crystallogr., 2003, 36, 7 CrossRef CAS.
- M. O'Keeffe, M. A. Peskov, S. Ramsden and O. M. Yaghi, Acc. Chem. Res., 2008, 41, 1782 CrossRef PubMed.
- M. D. Allendorf, C. A. Bauer, R. K. Bhakta and R. J. T. Houk, Chem. Soc. Rev., 2009, 38, 1330 RSC.
- P. Alama, G. Kaurb, C. Climentc, S. Pashaa, D. Casanovad, P. Alemanyc, A. R. Choudhuryb and I. R. Laskar, Dalton Trans., 2014, 43, 16431 RSC.
- A. Singh, T. Raj, T. Aree and N. Singh, Inorg. Chem., 2013, 52, 13830 CrossRef CAS PubMed.
- K. Jayaramulu, R. P. Narayanan, S. J. George and T. K. Maji, Inorg. Chem., 2012, 51, 10089 CrossRef CAS PubMed.
- Z. M. Hao, X. Z. Song, M. Zhu, X. Meng, S. N. Zhao, S. Q. Su, W. T. Yang, S. Y. Song and H. J. Zhang, J. Mater. Chem. A, 2013, 1, 11043 CAS.
- W. Liu, T. Jiao, Y. Li, Q. Liu, M. Tan, H. Wang and L. Wang, J. Am. Chem. Soc., 2004, 126, 2280 CrossRef CAS PubMed.
- L. E. Kreno, M. Allendorf and J. T. Hupp, Chem. Rev., 2012, 112, 1105 CrossRef CAS PubMed.
- Z. Y. Guo, H. Xu, S. Q. Su, J. F. Cai, S. Dang, S. C. Xiang, G. D. Qian, H. J. Zhang, M. O'Keeffe and B. L. Chen, Chem. Commun., 2011, 47, 5551 RSC.
- J. X. Ma, X. F. Huang, X. Q. Song and W. S. Liu, Chem.–Eur. J., 2013, 19, 3590 CrossRef CAS PubMed.
- S. Horike, S. Bureekaew and S. Kitagawa, Chem. Commun., 2008, 471 RSC.
- G. Y. Wang, C. Song, D. M. Kong, W. J. Ruan, Z. Chang and Y. J. Li, J. Mater. Chem. A., 2014, 2, 2213 CAS.
- H. Wang, W. Yang and Z. M. Sun, Chem.–Asian J., 2013, 8, 982 CrossRef CAS PubMed.
-
(a) B. Gole, A. K. Bar and P. S. Mukherjee, Chem. Commun., 2011, 47, 12137 RSC;
(b) S. Dang, E. Ma, Z. S. Sun and H. J. Zhang, J. Mater. Chem., 2012, 22, 16920 RSC;
(c) W. T. Yang, J. Feng and H. J. Zhang, J. Mater. Chem., 2012, 22, 6819 RSC;
(d) W. T. Yang, J. Feng, S. Y. Song and H. J. Zhang, ChemPhysChem, 2012, 13, 2734 CrossRef CAS PubMed;
(e) Y. Q. Xiao, L. B. Wang, Y. J. Cui, B. L. Chen, F. Zapata and G. D. Qian, J. Alloys Compd., 2009, 484, 601 CrossRef CAS.
- Q. R. Fang, D. Q. Yuan, J. L. Sculley, J. R. Li, Z. B. Han and H. C. Zhou, Inorg. Chem., 2010, 49, 11637 CrossRef CAS PubMed.
- S. C. Xiang, Y. B. He, Z. J. Zhang, H. Wu, W. Zhou, R. Krishna and B. L. Chen, Nat. Commun., 2012, 3, 954 CrossRef PubMed.
- Q. Tang, S. X. Liu, Y. W. Liu, J. Miao, S. J. Li, L. Zhang, Z. Shi and Z. P. Zheng, Inorg. Chem., 2013, 52, 2799 CrossRef CAS PubMed.
- C. Y. Sun, X. L. Wang, C. Qin, J. L. Jin, Z. M. Su, P. Huang and K. Z. Shao, Chem.–Eur. J., 2013, 19, 3639 CrossRef CAS PubMed.
- B. Adhikari, G. Palui and A. Banerjee, Soft Matter, 2009, 5, 3452 RSC.
- J. T. Jia, F. X. Sun, T. Borjigin, H. Ren, T. T. Zhang, Z. Bian, L. X. Gao and G. S. Zhu, Chem. Commun., 2012, 48, 6010 RSC.
- F. Pu, X. Liu, B. L. Xu, J. S. Ren and X. G. Qu, Chem.–Eur. J., 2012, 18, 4322 CrossRef CAS PubMed.
- H. L. Jiang, Y. Tatsu, Z. H. Lu and Q. Xu, J. Am. Chem. Soc., 2010, 132, 5586 CrossRef CAS PubMed.
- J. A. Greathouse, N. W. Ockwig, L. J. Criscenti, T. R. Guilinger, P. Pohl and M. D. Allendorf, Phys. Chem. Chem. Phys., 2010, 12, 12621 RSC.
- C. M. Doherty, Y. Gao, B. Marmiroli, H. Amenitsch, F. Lisi, L. Malfatti, K. Okada, M. Takahashi, A. J. Hill, P. Innocenzi and P. Falcaro, J. Mater. Chem., 2012, 22, 16191 RSC.
Footnote |
† Electronic supplementary information (ESI) available. CCDC 1437346. For ESI and crystallographic data in CIF or other electronic format see DOI: 10.1039/c6ra01709f |
|
This journal is © The Royal Society of Chemistry 2016 |
Click here to see how this site uses Cookies. View our privacy policy here.