DOI:
10.1039/C6RA07049C
(Paper)
RSC Adv., 2016,
6, 45708-45715
A reversible pH-modified fluorescence transition in block copolymer micelles enwrapped with a zinc(II) fluorescent complex†
Received
17th March 2016
, Accepted 15th April 2016
First published on 19th April 2016
Abstract
A reversible pH-modified blue to green luminescent transition system was constructed by encapsulating bis(2-(2-hydroxyphenyl)benzothiazolate) zinc (Zn(BTZ)2) within poly(styrene-b-acrylic acid) (PS-b-PAA) micelles. The system can respond to changes in the external pH environment from alkaline (7.0–10.5) to weakly acidic (4.5–7.0) conditions, which are absent for the pristine Zn(BTZ)2 dimethylformamide solution state, and designated as blue and green micelles, respectively. The tunable pH-modified luminescent behavior probably originates from changes in the Zn(BTZ)2 molecular packing modes modified by the shrink/swell morphology transition of the copolymer micelles due to the reversible ionization of the carboxylic group. The emission transition from blue to green can be further detected through affecting the Zn(BTZ)2 intermolecular interactions upon grinding the Zn(BTZ)2 powder. The micelles were further fabricated to form films by electrostatic layer-by-layer assembly with positively charged layered double hydroxide (LDH) nanosheets, which are responsive to acidic and alkaline atmospheres and show higher UV resistance compared to the micelles/PDDA (poly(dimethyldiallylammonium) chloride) film.
1. Introduction
Recently, stimuli-responsive block copolymers have received considerable attention, which typically undergo reversible or irreversible changes in physical or chemical properties upon an external stimulus (e.g. pH, temperature, light, pressure, atmosphere, or their combination).1–4 Their diverse applications ranging from chemosensors/biosensors to drug/dye delivery have been extensively explored.1,2,4 A current trend for pH-responsive sensors is to develop polymeric fluorescent probing systems (e.g. a thermoresponsive polymer labelled with disperse red 1 (ref. 4b) and block copolymer labelled with rhodamine1). Despite these remarkable advances, challenges remain. Copolymers must be grafted with fluorescent dye using covalent bonds, which usually involve a relatively time-consuming synthesis process. The fluorescent dye must be pH-sensitive, which might exhibit inherent limitations such as high background interference and less than satisfactory repeatability.2b On the other hand, it is generally recognized that the emission properties of metal luminophoric complexes strongly depend on the molecular arrangement motif and intermolecular interactions. Tuning and switching of the luminescence have been investigated based on some metal complexes (AuI, ZnII, PtII, IrIII, and AlIII), through an external stimulus such as grinding, which affects the mode of molecular packing.5 Amphiphilic block copolymers can form hydrophobic core/hydrophilic shell micelles in their aqueous solution, which can spontaneously enwrap small hydrophobic molecules.6 To the surprise, very few probing systems have been reported for core–shell micelles enwrapped metal complexes, which may be attributed to the fact that the bulky metal complexes are relatively difficult to assemble into the micelles when compared to small organic molecules. The construction of metal complex-based micelles would be beneficial to improve the stability of the micelle cores and access unique physical properties that are not possible from one component alone.7
Bis(2-(2-hydroxyphenyl)benzothiazolate)zinc(II) (Zn(BTZ)2), which exists as a monomeric or dimeric structure, has been widely investigated as an excellent white luminescent material in organic light-emitting diodes (OLEDs).8–10 A striking difference between their electroluminescent (EL) and photoluminescent (PL) spectra is observed.8b The EL spectra are strongly dependent on the thickness of the [Zn(BTZ)2]2 layer and the different viewing angles.8b,9 To date, the mechanism of the broad light emission observed for devices based on [Zn(BTZ)2]2 is indistinct and the optical interference effect or an exciplex formation is expected to be the main origin. Some new phenomena in regards with the emission features of [Zn(BTZ)2]2 have been discovered recently. Xu et al. achieved blue light emission by introducing different functional groups through complicated organic synthesis.10a,b Distinct differences in the molecular structures, such as molecular conformation or molecular packing, and their emission were observed upon introduction of methyl as a substituent.10c Due to the unique and tunable optical properties of the Zn(BTZ)2 complex, it may be possible to develop new types of luminescent sensor materials because the interaction and aggregation types of the complex can be adjusted by external stimuli, which can further change its emission properties. Furthermore, to achieve the requirements of luminescent devices or sensors, film systems with regular molecular orientation on two-dimensional surfaces are required.11 Layered double hydroxide (LDHs) are one important inorganic layered clay,12 widely used in catalysis, drug delivery and optical materials, which serve to enhance the thermal or optical stability of the guest within the LDH interlayer.13 Positively charged nanosheets exfoliated from LDHs14 can be used to fabricate functional films by electrostatic LbL deposition with many anionic species.11
We previously fabricated a tris(8-hydroxyquinoline-5-sulfonate)aluminum (AlQS) intercalated Mg–Al LDHs system with a cyan-blue luminescence transition, which is because of the isomerization of meridianal to facial AlQS via ligand flipping caused by a host–guest electrostatic interaction.13d A previous study also indicated that ordered films based on perylene/poly(N-vinyl carbazole) assembled with LDHs nanosheets displayed a reversible fluorescence response for volatile organic compounds due to 2D fluorescence resonance energy transfer.13h Herein, a reversible pH-modified fluorescence transition system based on Zn(BTZ)2@PS-b-PAA (poly(styrene-b-acrylic acid)) micelles was fabricated and developed. The micelles exhibit reversible blue/green photoluminescence (PL) responses to external pH. Their tunable pH-modified luminescent behavior, which are absent in the pristine Zn(BTZ)2 dimethylformamide (DMF) solution, can be confirmed to originate from the variational interactions of Zn(BTZ)2 molecules in the micelle core upon a morphology transition induced by a change in pH. The Zn(BTZ)2 powder also displays green light turning from blue light through grinding as a result of affecting the Zn(BTZ)2 intermolecular interactions. A supramolecular film based on the electrostatic layer-by-layer (LbL) assembly of micelles and positively charged LDH nanosheets was fabricated (see Scheme S1 in the ESI†). The (Zn(BTZ)2@PS-b-PAA/LDH)n films with good photo-stability also show reversible blue/green emission under an alternate NH3·H2O/HCl atmosphere.
2. Experimental
2.1 Reagents and materials
Bis[2-(2-benzothiazoly)phenolato]zinc(II) (C26H16N2O2S2Zn) was purchased from TCI (Shanghai) development Co., Ltd. Poly(tert-butyl acrylate-co-ethylacrylate-co-methacrylic acid), [CH2CH(CO2C(CH3)3)3]x[CH2CH(CO2C2H5)]y[CH2C(CH3)(CO2H)]z (PTBEM), and poly(dimethyldiallylammonium) chloride (PDDA, Mw = 100
000–200
000) were purchased from Sigma-aldrich development Co. Ltd. Amphiphilic diblock copolymer, poly(styrene-b-acrylic acid) (Mn: PS(12
000)-b-PAA(1100), Mw/Mn = 1.10; PS115–PAA15) was purchased from Polymer Source Inc. Analytical grade Mg(NO3)2·6H2O, Al(NO3)3·9H2O, NaOH, tetrahydrofuran (THF), methanol, N,N-dimethyl formamide (DMF) and formamide were purchased from Beijing Chemical Co. Ltd. All other chemicals were of analytical grade and used as received without further purification. The de-ionized water was used throughout the experimental processes.
2.2 Preparation of Zn(BTZ)2-micelles
(1) Preparation of the Zn(BTZ)2@PS-b-PAA micelles. (i) DMF was used as the solvent for PS-b-PAA: the preparation of Zn(BTZ)2 encapsulated within micelles was carried out according to a literature method with some modification.7d 5 mg of block polymer PS-b-PAA was dissolved in 1 mL of a 0.5 mg mL−1 Zn(BTZ)2 DMF solution and then 19 mL of de-ionized water was slowly added drop-wise under ultrasonication. The concentration of Zn(BTZ)2 in the Zn(BTZ)2@PS-b-PAA micelles solution was 0.025 mg mL−1, while that of PS-b-PAA was 0.25 mg mL−1. The pristine PS-b-PAA micelles were made using the same method with the exception being the absence of Zn(BTZ)2.(ii) THF was used as the solvent for PS-b-PAA: 5 mg of block copolymer PS-b-PAA was dissolved in 1 mL of THF and then 18 mL of de-ionized water was added dropwise under ultrasonication. Then, 1 mL of 0.5 mg mL−1 Zn(BTZ)2 methanol solution was injected slowly into the abovementioned PS-b-PAA micelles with ultrasonication.
(2) Preparation of the Zn(BTZ)2@PTBEM micelles. 7 mg of block copolymer PTBEM was dissolved in 18 mL of de-ionized water, the pH value was adjusted to pH 8.0 upon the addition of 0.1 M NaOH solution. Then, 2 mL of 0.5 mg mL−1 Zn(BTZ)2 DMF solution was injected slowly into the above PTBEM micelles under ultrasonication.
2.3 Fabrication of (Zn(BTZ)2@PS-b-PAA/LDH)n UTFs
The synthesis process and exfoliation of Mg–Al-LDH were similar to that described in previous study.18 0.1 g Mg–Al-LDH was shaken in 100 mL of formamide solution for 30 h to produce a colloidal suspension of exfoliated Mg–Al-LDH nanosheets. A quartz glass substrate was first cleaned in concentrated H2SO4/30% H2O2 (7
:
3) for 30 min. Then, the quartz substrate was washed thoroughly with de-ionised water. The cleaned substrates were dipped into a cationic PDDA solution (10 mg mL−1) for 15 min and then thoroughly rinsed with distilled water and dried under a stream of nitrogen gas, which resulted in a positively charged surface on the substrates. The pre-treated substrate was dipped in a solution of the Zn(BTZ)2@PS-b-PAA micelles at pH = 9.0 for 10 min followed by washing thoroughly. Then, the substrate was treated with a colloidal suspension (1 mg mL−1) of LDH nanosheets for 10 min and then washed thoroughly. The (micelles/LDH)n multilayer films were fabricated by alternately depositing the Zn(BTZ)2@PS-b-PAA micelles and LDH nanosheets suspension for n cycles. Every deposition was washed and dried under a stream of nitrogen gas.
Preparation of the (Zn(BTZ)2@PS-b-PAA/PDDA)n film was the same as that used to prepare the (Zn(BTZ)2@PS-b-PAA/LDH)n film with the exception that LDH nanosheets were replaced with a 10 mg mL−1 PDDA aqueous solution.
2.4 Characterization
11 UV-vis absorption spectra were obtained in the range from 190 to 700 nm on a Shimadzu U-3000 spectrophotometer with a slit width of 1.0 nm. Fluorescence spectra were performed on a RF-5301PC spectrophotometer. The reversible fluorescent measurement in an acidic/alkaline atmosphere was carried out as follows: (1) the film was hung and fixed in a quartz cell; (2) a few drops of 37 wt% HCl or 30 wt% NH3·H2O were alternately added to the bottom of the cell, so that the film can be exposed to an acidic/alkaline atmosphere, respectively, and then the fluorescence spectra were measured after 1–2 min; (3) before each measurement, the film was dried a few minutes at 40 °C. The 2D fluorescent spectra were obtained on a Hitachi F-7000 FL spectrophotometer with a slit width of 5 nm and a PMT voltage of 400 V. Steady-state polarized photoluminescence measurements were recorded on an Edinburgh Instruments' FLS 920 fluorometer and polarizers were used in the excited and detected directions. The fluorescence lifetime measurements were recorded using an Edinburgh Instruments' FLS 900 fluorometer with 375 nm excitation from a pulsed diode laser 800-B. The percentage contribution of each lifetime component to the total decay was calculated using the Edinburgh F900 instruments software. A Zeiss Supra 55 scanning electron microscope (SEM, the accelerating voltage was 20 kV, combined with energy-dispersive X-ray spectroscopy (EDX) for determination of Zn element), JEOL JEM-2010 high resolution transmission electron microscope (TEM, accelerating voltage was 200 kV) and NanoScope III Atomic Force Microscope (AFM, Veeco Instruments Co. Ltd.) were used to investigate the surface morphology of the samples. The zeta-potential measurement and dynamic lighting scattering (DLS) diameter were conducted using a Malvern instrument (Nano ZS90) equipped with a 632.8 nm He–Ne laser. CPTOSS solid-state NMR spectra were obtained using a Bruker AVANCE III 400 WB at 20 °C at 4 kHz spinning.
3. Results and discussion
3.1 The structure and morphology of the Zn(BTZ)2@PS-b-PAA micelles
Scheme 1 shows a representation of the core/shell Zn(BTZ)2@PS-b-PAA micelles at different pH values. PS-b-PAA, which can easily form negatively charged micelles, was chosen to incorporate the Zn(BTZ)2 molecules based on hydrophobic interactions.7 When the pH was in the range of 7.0–10.5, the micelles show blue luminescence under 365 nm UV irradiation. After the pH was changed to 4.5–7.0, they instantly produce a green luminescence. Inset (a), (b) and (c) of Scheme 1 show the luminescence transition images of the micelles at pH = 6.0 and 9.0. The Zn(BTZ)2@PS-b-PAA micelles exhibited the Tyndall effect (see Fig. S1 in the ESI†) were proved to be stable and of engineering robustness, which allowed for the following LbL fabrication.7d The Zn(BTZ)2 encapsulated within the PS-b-PAA micelles was proved by scanning electron microscopy-energy dispersive X-ray fluorescence analysis (SEM-EDX), which detected the existence of the Zn element (see Fig. S2 in the ESI†). Transmission electron microscopy (TEM) displays the Zn(BTZ)2 molecules distributing the peripheral part inside the micelle core (see Fig. S3 in the ESI†). The SEM images (Fig. 1a–c) reveal that an individual micelle has a spherical morphology and was around 20, 35, 60 nm in diameter at pH = 6.0, 7.5, and 9.0, respectively, and was also confirmed by their atomic force microscope (AFM) images (see Fig. S4 in the ESI†). The spherical micelles prefer to aggregate into a “pearl-necklace-like” morphology at pH = 6.0 for the hydrogen bonding interactions between the individual micelle (Fig. 1a).15 The dynamic lighting scattering (DLS) results (Fig. 1d and S5 in the ESI†) indicate that the micellar diameter (DDLS) were centered at 35, 40, and 51 nm at pH = 6.0, 7.5 and 9.0, respectively, which is consistent with the increasing trend in diameter observed by SEM and AFM. Moreover, the zeta-potential value of micelles undergoes an obvious decrease from −17.4 to −51.6 mV upon increasing the pH value from 6.0 to 9.0, indicating that a higher degree of ionization of PS-b-PAA at higher pH leads to the expansion of micelles (Fig. 1d and S5 in the ESI†). These observations illustrate the existence of pH-driven morphological transitions in the Zn(BTZ)2@PS-b-PAA micelles. For comparison, PS-b-PAA micelles were also characterized. The SEM diameters and ζ-potential value of the PS-b-PAA micelles at pH = 6.0, 7.5 and 9.0 exhibit weaker similar variations of (25 nm, −12.4 mV), (28 nm, −15.3 mV) and (33 nm, −17.4 mV), respectively, which are not shown here. The encapsulation of small amount of Zn(BTZ)2 causes the expansion of the micelle size and an enhanced ionization of the peripheral carboxyl group in the block copolymer micelles with an unconsidered morphological change.7c From a comparison of the zeta-potential value of the PS-b-PAA micelles at varying pH, the existence of Zn(BTZ)2 was inferred to accelerate the ionization of the PAA group and the reason for this still needs further investigation.
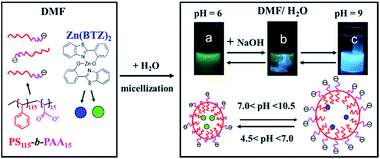 |
| Scheme 1 Representations of the core/shell Zn(BTZ)2@PS-b-PAA micelles at different pH values. (a) and (c) Images of micelles at pH = 6.0 and 9.0 under 365 nm UV light irradiation. (b) The transition process from green to blue light after increasing the pH value in (a). | |
 |
| Fig. 1 The SEM morphology of Zn(BTZ)2@PS-b-PAA micelles at pH (a) 6.0, (b) 7.5 and (c) 9.0. (d) Zeta-potential and DLS diameter as a function of pH value for the Zn(BTZ)2@PS-b-PAA micelles. | |
3.2 The pH-modified photophysical properties of the Zn(BTZ)2@PS-b-PAA micelles
To obtain insight into the PL change of encapsulated Zn(BTZ)2 dependent on the external pH values, their photophysical properties were studied. The fluorescence emission spectra shown in Fig. 2a are centered at 510 and 460 nm for the micelles at pH = 6.0 and 9.0, respectively, while the micelles at pH = 6.0 and 9.0 exhibit one distinct main excitation spectra around 340 and 390 nm, respectively. The micelles at pH = 7.5 exhibit a dual emission at 460 and 510 nm (see Fig. S6 in the ESI†). The 2D fluorescence spectra of the Zn(BTZ)2@PS-b-PAA micelles further display that the emission peak was mainly located at 510 nm at pH = 6.0, while blue-shifted to 460 nm (ligand-based π–π* transitions8b) at pH = 9.0 (Fig. 2b and c) with a wide excitation.
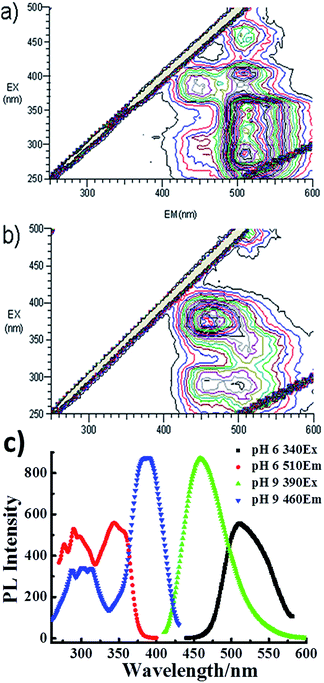 |
| Fig. 2 3D fluorescence spectra of the Zn(BTZ)2@PS-b-PAA micelles at (a) pH = 6.0 and (b) pH = 9.0. (c) Fluorescence emission and excitation spectra of the micelles at pH = 6.0 and pH = 9.0, respectively. | |
Therefore, the PL for the Zn(BTZ)2@PS-b-PAA micelles at pH = 6.0 and 9.0 under excitation at 340 and 390 nm were emphasized. A Zn2(4-tfmBTZ)4/tetrahydrofuran (THF) solution was also observed to display a image discrepancy with excitation spectra due to the possible existence of an oligomer.10a The remarkable difference can be further directly observed from the images of the micelles at pH = 6.0 and 9.0 under 365 nm UV irradiation (Scheme 1a and c, inset), which exhibit visible green and blue luminescence, respectively. Nevertheless, the pH-modified luminescence properties are absent for the Zn(BTZ)2 DMF solution with 467 nm emission (see Fig. S7 in the ESI†). Therefore, this means the pH-modified luminescence behavior possibly originates from changes in the microenvironment and polarity of Zn(BTZ)2, and the mode of molecular packing modified by the morphological transitions in the PS-b-PAA micelles. At pH = 6.0, the degree of ionization of PS-b-PAA is ca. 30%, and thus, 70% of the unionized carboxylate (COOH) group go inwards into the micelle core, which will be close to the Zn(BTZ)2 molecules.7e The degree of ionization of PS-b-PAA increased to ca. 73% and 90% at pH = 7.5 and 9.0, respectively, thus, there are 27% and 10% COOH close to the Zn(BTZ)2.7e This is determined to be the reason for the change in the microenvironment and core polarity of Zn(BTZ)2, which will further influence their emission. Lots of studies report that the emission spectra of a number of transition metal complexes is highly dependent on solvent polarity.16
The abovementioned assumption in regard the change in microenvironment and mode of molecular packing of Zn(BTZ)2 modified by pH could be evidenced by its fluorescence lifetime and polarization. The precise nature of the fluorescence decay can reveal details about the interactions of the fluorophores with their environment. The fluorescence lifetime of the micelles at pH = 6.0 is a little shorter than at pH = 9.0 (3.73 vs. 4.06 ns), which are all shorter than 4.53 ns observed for the Zn(BTZ)2 DMF solution, suggesting the Zn(BTZ)2 was in a different environment (Table S1 and Fig. S8 in the ESI†). This was also observed for the lifetime of the ultrathin film (UTF)-n under an alkaline atmosphere (the detail of the fabrication of the film will be discussed later), which turns from 3.36 to 2.96 ns after exposure to an HCl atmosphere. Polarized luminescence was employed to probe the influence of the Zn(BTZ)2 arrangement to the anisotropy value r of Zn(BTZ)2 in micelles.17 The micelles at pH = 6.0 with a diameter of 20 nm show a uniform r value of ca. 0.30, while r = 0.05 for micelles at pH = 9.0 with a diameter 60 nm (Fig. 3), indicating that the Zn(BTZ)2 molecules arrange with more order in the smaller micelles in an acidic environment than they do in the larger ones under an alkaline environment. In contrast to the Zn(BTZ)2 DMF solution with no anisotropy (r = 0.05) (see Fig. S9 in the ESI†), it can be concluded that in the larger micellar core under alkali conditions, the Zn(BTZ)2 molecules are possibly free, analogous to that in the DMF solution.
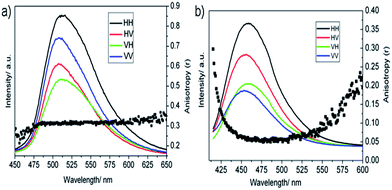 |
| Fig. 3 Polarized fluorescence profiles in the HH, HV, VV, VH modes and anisotropic value (r) for Zn(BTZ)2@PS-b-PAA micelles at (a) pH 6.0 under 340 nm excitation and (b) pH 9.0 under 390 nm excitation. | |
Good reversibility and stability are important for the practical applications of pH-modified luminescence materials. The transition from green to blue emission of the micelles is instant and can be readily repeated at least 80 times. The green/blue micelles are stable for more than 30 days (Fig. S10 ESI†). Furthermore, by replacing PS-b-PAA and the DMF solvent with another block copolymer PTBEM13g (Fig. 4) and THF (see Fig. S11 in the ESI†), they exhibit nearly the same pH-modified luminescence behavior as that of the Zn(BTZ)2@PS-b-PAA micelles, demonstrating the generality of the pH-modified luminescence effect for the Zn(BTZ)2@block copolymer micelles.
 |
| Fig. 4 PL emission spectra of the Zn(BTZ)2@PTBEM micelles at pH 6.0 (a) and 9.0 (b) under 340 and 390 nm excitation, respectively. | |
3.3 The similar luminescence transition observed when grinding Zn(BTZ)2
The transition of blue to green luminescence is also detected when grinding the Zn(BTZ)2 powder. Upon harsh grinding with a pestle, the blue luminescent Zn(BTZ)2 powders transfer to green emissive solids (Fig. 5 inset). The luminescence transformation could be distinctly observed from the spectral difference (Fig. 5). The fluorescence spectrum of the Zn(BTZ)2 powder was observed with a mono-emission peak at 470 nm, whereas an emission centered at 483 nm is shown on the ground powder, agreeing well with the color changes of the Zn(BTZ)2 solids when treated with grinding (Fig. 5a and b). However, the ground Zn(BTZ)2 green powder can quickly turn to blue after dissolving into a DMF solution. For comparison, the spectra of the ground Zn(BTZ)2 powder dissolved in DMF solution blue shifts back to 462 nm (Fig. 5d), which is similar to the Zn(BTZ)2 DMF solution located at 467 nm (Fig. 5c). A lot of metal complexes (such as iridium(III) complexes and tris(8-hydroxyquinoline)aluminium) undergo remarkable emission color changes upon grinding, which are caused by affecting the mode of molecular packing through an external stimulus.5
 |
| Fig. 5 Emission spectra at 340 nm excitation of (a) Zn(BTZ)2 powder, (b) ground Zn(BTZ)2 powder, (c) Zn(BTZ)2 powder and (d) ground Zn(BTZ)2 powder dissolved into DMF solution; the top shows Zn(BTZ)2 molecules changing from blue to green after grinding under 365 nm UV light irradiation. | |
To determine if the possible mechanism of the pH-modified luminescent behavior maybe originating from the rearrangement of the Zn(BTZ)2, powder X-ray diffraction (XRD) measurements and solid nuclear magnetic resonance (NMR) were performed on Zn(BTZ)2 and the ground Zn(BTZ)2 powder. Intensive and sharp reflection peaks are observed in the XRD patterns for Zn(BTZ)2, while they turn to be weak and broad for the ground Zn(BTZ)2 powder, as shown in Fig. S12 in the ESI. † The results indicated that the crystalline Zn(BTZ)2 powders with a well-organized structure were disrupted into amorphous states after grinding, together with the variation of molecular stacking motif, which is also reported for ground crystalline Alq3.5e A correlation between the molecular stacking structure and optical properties also happened in methyl-substituted Zn(BTZ)2 derivatives through C–H⋯S hydrogen bonds interactions.10a,c In addition, intermolecular interactions between adjacent BTZ ligands may be a key factor that affects the luminescence of the blue and green Zn(BTZ)2 solids.
To investigate the variation of the intermolecular interactions before and after grinding, CPTOSS 13C NMR of Zn(BTZ)2 and its ground samples was carried out and proved to be especially efficient in disclosing the information related to the intermolecular interactions between the adjacent ligands (Fig. S13 in the ESI†). The observed 13C resonances for Zn(BTZ)2 powder were sharp with 13 signals, δ = 113.8, 117.2, 120.3, 121.8, 125.1, 128.4, 130.1, 132.1, 150.9, 152.9, 161.8, 168.5 and 175.7 ppm, which is equal to the number of C in the BTZ ligand. An effect of the intermolecular interactions could be obviously observed from the shift of all the 13C resonances for the ground Zn(BTZ)2 powder to a lower frequency of about 0.4 ppm between 113.5 and 175.3 ppm. In contrast to our traditional knowledge that Zn(BTZ)2 are blue emissive, we proved the existence of the green emission Zn(BTZ)2 for the first time and disclose that intermolecular interaction may be a key factor that determines the solid luminescence of Zn(BTZ)2 based on NMR data and the comparison of the excitation spectra (Fig. 5). The swollen/shrinking micellar core size upon variation of the micellar pH may cause the rearrangement of Zn(BTZ)2 stacking, which is reflected in the emission transition of the Zn(BTZ)2@PS-b-PAA micelles. It is probably similar with the present piezochromic behavior of Zn(BTZ)2.
3.4 The pH-modified photophysical properties of the (Zn(BTZ)2@PS-b-PAA micelles/LDH)n films
To further study and apply the pH-modified luminescence micelles, an ultrathin film (UTF) containing the Zn(BTZ)2@PS-b-PAA micelles was further constructed. The subsequent assembly process for the (micelles/LDH)n films (micelles represent Zn(BTZ)2@PS-b-PAA at pH = 9.0, UTF-n) was monitored by UV-visible absorption and PL spectra (Fig. 6). The absorption band intensities at 220 nm (π–π* transition of phenyl ring in the PS block and Zn(BTZ)2 (ref. 8b)) correlate linearly with the bilayer number, n (Fig. 6a, inset), demonstrating a stepwise and regular Zn(BTZ)2 deposition and film growth procedure, which can be visually demonstrated by images of the UTFs under UV-lamp irradiation (Fig. 6, inset). The PL intensities at 450 nm under 390 nm excitation present a consistent enhancement with an increase in n (Fig. 6b).
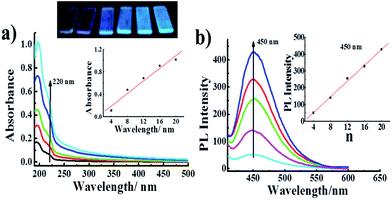 |
| Fig. 6 (a) UV-vis absorption and (b) emission spectra at 390 nm excitation of UTF-n (n = 4–20). Inset in (a) absorbance at 220, and (b) emission at 450 nm vs. n, as well as images of the UTFs under UV irradiation. | |
The UTF-n exhibits similar excitation and emission spectra to those observed for Zn(BTZ)2@PS-b-PAA micelles at pH = 9.0. There are no significant morphology changes of the micelles assembled into the UTF-n when compared to the Zn(BTZ)2@PS-b-PAA micelles at pH = 9.0 with diameter of 60 nm, suggesting that the micelle structure can remain within the LDH interlayer. The surface coverage of micelles adsorbed on the substrate increased with n (see Fig. S14 in the ESI†).
The UTF-n undergoes a significant luminescence transition under a HCl or NH3·H2O atmosphere for a short period (1–2 min), as shown in Fig. 7. When exposing the UTF-16 film to an HCl atmosphere, the 450 nm emission is significantly weakened and the 505 nm emission enhanced, thus leading to an increase in the intensity ratio of I505/I450 for the 340 nm excitation, which is similar to those observed for the micelles at pH = 9.0 to 6.0. The PL transition can be repeated by alternative exposure to a NH3·H2O or HCl atmosphere (Fig. 7). Although the HCl atmosphere will cause some damage to the LDH layer, the UTF-n still shows a fatigue resistance to some extent. The reversible luminescence conversion of the film was further visible from the uniform blue to green color (see inset in Fig. 7). The micelles diameter changes from 60 nm to 30 nm, as shown in the top view SEM (Fig. 8a and b), together with the thickness per (micelles/LDH)1 inferred from cross-sectional SEM images transforms from ca. 48 nm to 30 nm after exposure to a HCl atmosphere for 2 min (Fig. 8c and d).
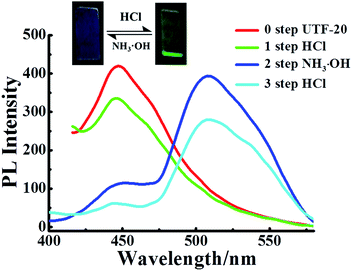 |
| Fig. 7 Reversible luminescence transition of UTF-16 alternately exposing to HCl and NH3·H2O atmosphere with excitation at 340 (in alkaline condition) and 390 nm (in weak acidic condition). Inset: photographs taken under 365 nm UV light. | |
 |
| Fig. 8 SEM top view images of (a) (micelles/LDH)16 film with micelles as the outer layer and (b) the film exposed to a HCl atmosphere for 2 min. Cross-sectional SEM images of (c) UTF-16 and (d) UTF-16 exposed to a HCl atmosphere. | |
3.5 The photostability of the (micelles/LDH)n films
Good photo-stability is an extremely important criterion for the practical applications of the as-prepared thin films. In situ photoluminescence spectra measurements were performed to compare the optical stability of the UTF-n with other micelles/polycation systems. Herein, poly(dimethyldiallylammonium chloride) (PDDA) was chosen as the commutative polycation to obtain the (micelles/PDDA)n UTF. The absorption band intensities at 340 nm and their emission intensities at 460 nm present consistent enhancement with an increase in n (see Fig. S15 in the ESI†), demonstrating a stepwise and regular Zn(BTZ)2 deposition and film growth procedure. After continuous irradiation with 390 nm UV light for 5 min, the intensity decreases by ca. 30% and 10% for (micelles/PDDA)16 and UTF-16, respectively. Furthermore, the luminescence intensity of the UTF decreases gradually by 15% in the time range of 0–40 min, while the (micelles/PDDA)n films decrease quickly by 55% within the first 35 min (Fig. 9). It can be concluded that the UTF based on LDH presents improved UV-light resistance ability compared to the traditional film assembled micelles with other polycations such as (micelles/PDDA)n film.
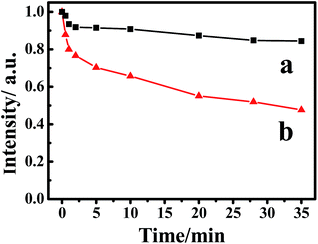 |
| Fig. 9 The decay of the normalized maximal PL intensity with time, normalized against the initial PL value; λex = 390 nm to probe the UV irradiation resistance ability of (micelles/LDH)16 (curve a) and the (micelles/PDDA)16 (curve b) film. | |
4. Conclusions
In summary, unlike the pristine Zn(BTZ)2 DMF solution without fluorescent transition properties, the pH-modified luminescent behavior and reversible green/blue emission change upon varying the acid/alkali atmosphere for Zn(BTZ)2 encapsulated within PS-b-PAA micelles that were assembled into a UTF-n with LDH nanosheets has been demonstrated. The abovementioned transition probably originates from the changes in the Zn(BTZ)2 molecular packing modes, which is quite similar to the piezochromic behavior of Zn(BTZ)2 powder. The micelles show good reversibility and stability. The assembly of the micelles and LDH nanosheets into the UTF-n leads to a higher UV photostability for Zn(BTZ)2. The reversible pH-modified luminescence effect suggests they have potential applications as luminescent sensors or switches. It can be anticipated that this approach based on the encapsulation of a neutral complex into block copolymer micelles can also be effectively utilized to fabricate other pH-modified luminescence materials.
Acknowledgements
This study was supported by the National Natural Science Foundation of China, 111 Project (Grant No. B07004), New Century Excellent Talents in University (NCET-11-0560) and the Fundamental Research Funds for the Central Universities.
Notes and references
- K. Zhou, Y. Wang, X. Huang, K. Luby-Phelps, B. D. Sumer and J. Gao, Angew. Chem., Int. Ed., 2011, 50, 6109 CrossRef CAS PubMed.
-
(a) C. Li, Y. Zhang, J. Hu, J. Cheng and S. Liu, Angew. Chem., Int. Ed., 2010, 49, 5120 CrossRef CAS PubMed;
(b) J. Hu, X. Zhang, D. Wang, X. Hu, T. Liu, G. Zhang and S. Liu, J. Mater. Chem., 2011, 21, 19030 RSC.
- H. Lee, W. Wu, J. K. Oh, L. Mueller, G. Sherwood, L. Peteanu, T. Kowalewski and K. Matyjaszewski, Angew. Chem., Int. Ed., 2007, 46, 2453 CrossRef CAS PubMed.
-
(a) W. Chen, Y. Cheng and B. Wang, Angew. Chem., Int. Ed., 2012, 51, 5293 CrossRef CAS PubMed;
(b) C. Pietsch, R. Hoogenboom and U. S. Schubert, Angew. Chem., Int. Ed., 2009, 48, 5653 CrossRef CAS PubMed.
-
(a) H. Ito, T. Saito, N. Oshima, N. Kitamura, S. Ishizaka, Y. Hinatsu, M. Wakeshima, M. Kato, K. Tsuge and M. Sawamura, J. Am. Chem. Soc., 2008, 130, 10044 CrossRef CAS PubMed;
(b) S. Mizukami, H. Houjou, K. Sugaya, E. Koyama, H. Tokuhisa, T. Sasaki and M. Kanesato, Chem. Mater., 2005, 17, 50 CrossRef CAS;
(c) T. Abe, T. Itakura, N. Ikeda and K. Shinozaki, Dalton Trans., 2009, 711 RSC;
(d) G. Shan, H. Li, H. Cao, D. Zhu, P. Li, Z. Su and Y. Liao, Chem. Commun., 2012, 48, 2000 RSC;
(e) H. Bi, D. Chen, D. Li, Y. Yuan, D. Xia, Z. Zhang, H. Zhang and Y. Wang, Chem. Commun., 2011, 47, 4135 RSC.
- S. A. Jenekne and X. L. Chem, Science, 1998, 279, 1903 CrossRef.
-
(a) Y. Kang and T. A. Taton, Angew. Chem., Int. Ed., 2005, 44, 409 CrossRef CAS PubMed;
(b) J. Cho, J. Hong, K. Char and F. Caruso, J. Am. Chem. Soc., 2006, 128, 9935 CrossRef CAS PubMed;
(c) N. Ma, Y. Wang, Z. Wang and X. Zhang, Langmuir, 2006, 22, 3906 CrossRef CAS PubMed;
(d) N. Ma, H. Zhang, B. Song, Z. Wang and X. Zhang, Chem. Mater., 2005, 17, 5065 CrossRef CAS;
(e) J. Choi and M. F. Rubner, Macromolecules, 2005, 38, 116 CrossRef CAS.
-
(a) G. Yu, S. Yin, Y. Liu, Z. Shuai and D. Zhu, J. Am. Chem. Soc., 2003, 125, 14816 CrossRef CAS PubMed;
(b) X. Xu, Y. Liao, G. Yu, H. You, C. Di, Z. Su, D. Ma, Q. Wang, S. Li, S. Wang, J. Ye and Y. Liu, Chem. Mater., 2007, 19, 1740 CrossRef CAS.
- M. Qureshi, S. S. Manoharan, S. P. Singh and Y. N. Mahapatra, Solid State Commun., 2005, 133, 305 CrossRef CAS.
-
(a) H. Xu, Y. Yue, H. Wang, L. Chen, Y. Hao and B. Xu, J. Lumin., 2012, 132, 919 CrossRef CAS;
(b) H. Xu, H. Wang, Y. Yue, L. Chen, Y. Hao and B. Xu, Synth. Met., 2012, 162, 775 CrossRef CAS;
(c) H. Xu, B. Xu, X. Fang, L. Chen, H. Wang and Y. Hao, J. Photochem. Photobiol., A, 2011, 217, 108 CrossRef CAS.
-
(a) D. P. Yan, J. Lu, M. Wei, J. B. Han, J. Ma, F. Li, D. G. Evans and X. Duan, Angew. Chem., Int. Ed., 2009, 121, 3119 CrossRef;
(b) D. P. Yan, J. Lu, J. Ma, M. Wei, D. G. Evans and X. Duan, Angew. Chem., Int. Ed., 2011, 50, 720 CrossRef CAS PubMed;
(c) D. P. Yan, J. Lu, J. Ma, S. Qin, M. Wei, D. G. Evans and X. Duan, Angew. Chem., Int. Ed., 2011, 50, 7037 CrossRef CAS PubMed.
-
(a) X. Duan and D. G. Evans, Chem. Commun., 2006, 5, 485 Search PubMed;
(b) Q. Wang and D. O'hare, Chem. Rev., 2012, 112, 4124 CrossRef CAS PubMed;
(c) F. Leroux and C. Taviot-Gueho, J. Mater. Chem., 2005, 15, 3628 RSC.
-
(a) L. He, Y. Huang, A. Wang, X. Wang, X. Chen, J. J. Delgado and T. Zhang, Angew. Chem., Int. Ed., 2012, 51, 6191 CrossRef CAS PubMed;
(b) T. Chen, F. Zhang and Y. Zhu, Catal. Lett., 2013, 143, 206 CrossRef CAS;
(c) J.-H. Choy, S.-Y. Kwak, Y.-J. Jeong and J.-S. Park, Angew. Chem., Int. Ed., 2000, 39, 4042 CrossRef CAS;
(d) S. Li, J. Lu, M. Wei, D. G. Evans and X. Duan, Adv. Funct. Mater., 2010, 20, 2848 CrossRef CAS;
(e) S. Li, J. Lu, J. Xu, S. Dang, D. G. Evans and X. Duan, J. Mater. Chem., 2010, 20, 9718 RSC;
(f) S. Li, J. Lu, H. Ma, J. Xu, D. Yan, M. Wei, D. G. Evans and X. Duan, Langmuir, 2011, 27, 11501 CrossRef CAS PubMed;
(g) S. Li, J. Lu, H. Ma, D. Yan, Z. Li, S. Qin, D. G. Evans and X. Duan, J. Phys. Chem. C, 2012, 116, 12836 CrossRef CAS;
(h) Z. Li, J. Lu, S. Li, S. Qin and Y. Qin, Adv. Mater., 2012, 24, 6053 CrossRef CAS PubMed.
- Z. P. Liu, R. Z. Ma, M. Osada, N. Iyi, Y. Ebina, K. Takada and T. Sasaki, J. Am. Chem. Soc., 2006, 128, 4872 CrossRef CAS PubMed.
- S. Yang, X. Yu, L. Wang, Y. Tu, J. X. Zheng, J. Xu, R. M. Van Horn and S. Z. D. Cheng, Macromolecules, 2010, 43, 3018 CrossRef CAS.
-
(a) A. Beeby, S. Bettington, I. D. W. Samuel and Z. Wang, J. Mater. Chem., 2003, 13, 80 RSC;
(b) J. N. Demas and B. A. DeGraff, Coord.
Chem. Rev., 2001, 211, 317 CAS.
- B. Valeur, Molecular Fluorescence: Principles and Applications, Wiley-VCH, Berlin, Germany, 2001, vol. 125, ch. 5 Search PubMed.
- J. B. Han, J. Lu, M. Wei, Z. L. Wang and X. Duan, Chem. Commun., 2008, 41, 5188 RSC.
Footnote |
† Electronic supplementary information (ESI) available: A schematic representation of the formation of PS-b-PAA@Zn(BTZ)2 micelles and fabrication of the (micelles/LDH)n films, Tyndall effect, SEM-EDX analysis, TEM image, AFM images of the Zn(BTZ)2@PS-b-PAA micelles. Zeta-potential and dynamic lighting scattering (DLS) diameter as a function of the pH value of the Zn(BTZ)2@PS-b-PAA micelles. Fluorescence decay profile for Zn(BTZ)2 DMF solution, micelles and film. Polarized fluorescence profiles and anisotropic value for the Zn(BTZ)2 DMF solution. Reversibility transition from green to blue emission of Zn(BTZ)2@PS-b-PAA micelles repeated for 20 cycles; (b) the PL intensity of Zn(BTZ)2@PS-b-PAA micelles (stored at room temperature) recorded for 32 days. Powder X-ray diffraction patterns and CPTOSS 13C NMR spectra of the Zn(BTZ)2 molecules before and after grinding. The absorption and PL emission spectra of (micelles/PDDA)n. See DOI: 10.1039/c6ra07049c |
|
This journal is © The Royal Society of Chemistry 2016 |
Click here to see how this site uses Cookies. View our privacy policy here.