DOI:
10.1039/C6RA09344B
(Paper)
RSC Adv., 2016,
6, 62550-62555
Synthesis of sub-100 nm biocompatible superparamagnetic Fe3O4 colloidal nanocrystal clusters as contrast agents for magnetic resonance imaging†
Received
11th April 2016
, Accepted 23rd June 2016
First published on 24th June 2016
Abstract
Synthesis of magnetic particles with superparamagnetism, high magnetization, excellent biocompatibility and small particle size is highly desirable for biomedical applications. Herein, we report that sub-100 nm biocompatible Fe3O4 nanocrystal clusters can be prepared via a solvothermal method by using water as a size-control agent and sodium citrate as a stabilizer. The resultant Fe3O4 particles have uniform spherical shape, superparamagnetic properties, high magnetization values, excellent colloidal stability and good biocompatibility. Notably, the particle size can be tuned over a range of 70–180 nm by changing the content of H2O in the system. Moreover, the T2-weighted magnetic resonance imaging (MRI) experiments show that 85 nm Fe3O4 particles have an ultra-high r2 relaxivity of 175.4 mM−1 s−1, suggesting a great potential as MRI probes. In addition, the labeling efficiency of mesenchymal stem cells with as-obtained Fe3O4 particles is much higher than that of commercial superparamagnetic iron oxide nanoparticles, which further indicates their promising in biomedicine.
Introduction
Magnetic nanoparticles, especially iron oxides, have attracted much attention in the past decades owing to their unique magnetic properties and promising application in catalysis, separation and biomedicine.1–5 For biomedical applications in molecular imaging of tumors,6–15 drug delivery,16–21 and hyperthermia treatment,22–24 many efforts have been devoted to control over the particle size, size distribution and surface property of iron oxide nanoparticles because these parameters strongly determine the physiochemical properties of magnetic nanoparticles and their pharmacokinetic behaviors as well.25–28 It is known that Fe3O4 particles would show superparamagnetic characteristic at room temperature when the particle size decreases to a small value (<30 nm), which are not susceptible to strong magnetic interaction on each other, favoring their stability in physiological solution.
Up to date, a large number of methods have been developed to synthesize superparamagnetic magnetite nanocrystals.29–32 For example, thermal decomposition synthesis, through the pyrolysis of Fe organometallic precursors in nonpolar solution, has been demonstrated to be one of the most effective routes to fabricate monodisperse magnetic nanocrystals with high crystallinity and small particle size (<20 nm).33–35 However, the additional surface hydrophilic/biocompatible modification and the weak magnetic responsiveness in solution hamper their practical use in bio-related applications, which is also a critical and common issue in co-precipitation, sol–gel synthesis and hydrothermal synthesis methods. With the aim of increasing the magnetic responsiveness in a controllable manner, several methods have been reported to assemble primary magnetite nanocrystals into secondary large particles, which can well retain the superparamagnetic properties, whereas a single-crystalline magnetite particle within the same size range would show ferromagnetic characteristic. For example, emulsion approaches have been explored to form aggregates of Fe3O4 nanocrystals.36 However, it needs strict reaction conditions and an outer shell to protect/modify the obtained the magnetic nanoparticles. Recently, one-step solvothermal method has been demonstrated as a potent, facile and time-saving method for preparation of magnetic nanocrystal clusters. Deng et al. first reported the solvothermal approach to synthesize 200–800 nm magnetite particles by reduction of FeCl3 with ethylene glycol.37 By introducing poly(acrylic acid) (PAA) as a stabilizer, the particle size of the water-dispersible superparamagnetic nanocrystal clusters (SPNCs) can decrease to 30–180 nm, but the polyelectrolyte PAA attached on the magnetic clusters is not biodegradable and biocompatible.38 Zhao and co-workers described that SPNCs with excellent biocompatibility and colloidal stability can be synthesized by using sodium citrate as the stabilizer, which has been widely used for synthesis of more than 180 nm SPNCs.39 Despite these promising achievements, it is still a great challenge to synthesize SPNCs with excellent biocompatibility, high mass magnetization and small particle size less than 100 nm, which has been proved to favor the bio-distribution and the enhanced permeability retention (EPR)-enhanced target delivery.40,41
Herein, we report, for the first time, the synthesis of sub-100 nm biocompatible SPNCs via a facile solvothermal method by using water as a size-control agent. The as-obtained SPNCs have uniform and tunable size in range from 180 to 70 nm by increasing the content of H2O from 0 to 2.5 mL, and more importantly show superparamagnetic behavior, high magnetization, excellent water dispersibility and low cytotoxicity. We explore their applicability in bio-medicine, which show an ultra-high transverse relaxivity (r2 = 175.4 mM−1 s−1 for 85 nm SPNCs) and can be used to efficiently label mesenchymal stem cells, much superior to commercial superparamagnetic iron oxide nanoparticles (SPIOs).
Experimental section
Materials
FeCl3·6H2O, trisodium citrate, sodium acetate, ethylene glycol, and ethanol were of analytical grade and purchased from the Shanghai Chemical Corp (Shanghai, China). Dulbecco's modified eagle medium (DMEM), heat-inactivated fetal bovine serum (FBS), phosphate buffered solution (PBS), dimethyl sulfoxide (DMSO), and penicillin–streptomycin solution were bought from Gibco/Life Technologies (Grand Island, New York, USA). Paraformaldehyde was obtained from KeyGEN Biotech Co., Ltd. (Jiangsu, China). 3-(4,5-Dimethylthiazol-2-yl)-2,5-diphenyltetrazolium bromide (MTT) was purchased from Nanjing Keygen Biotech. Co., Ltd. (Nanjing, China). The human breast cell MCF-7 cell line and MSCs were obtained from American Type Culture Collection (ATCC). Deionized water (Millipore, ≥18 MΩ cm) was used in all experiments.
Synthesis of colloidal superparamagnetic nanocrystal clusters
The SPNCs were synthesized via a solvothermal reaction by using citrate as the stabilizer and water as a size-control agent. Typically, 3.25 g FeCl3·6H2O, 1.3 g trisodium citrate, 6.0 g sodium acetate and H2O were in order dissolved in 100 mL ethylene glycol. The mixture was stirred vigorously for more than 1 h to ensure them dissolve thoroughly. The solution was then transferred to Teflon-lined stainless-steel autoclaves (25 mL) and heated to 200 °C for 10 h. The black product was collected by a magnet and washed with water thoroughly. To synthesize SPNCs with different sizes, the volume of water was varied from 0, 1.0, 1.5, 2.0, to 2.5 mL.
Characterization
Scanning electron microscopy (SEM) images were obtained on a Philips XL30 electron microscope (Netherlands) operated at 20 kV. Transmission electron microscopy (TEM) images were taken with a JEOL 2011 microscope (Japan) operated at an accelerating voltage of 100 kV. The samples were dispersed ultrasonically in ethanol and dropped on a carbon-film-coated copper grid for analysis. X-ray power diffraction (XRD) was performed using a D8 Focus Diffractometer (Bruker) with Cu Ka radiation. Magnetic characterization was recorded on a Quantum Design MPMS-XL SQUID magnetometer. The Fourier Transform Infrared Spectroscopy (FT-IR) spectra were recorded on a Perkin-Elmer 580B IR spectrophotometer using the KBr pellet technique. Zeta potential were measured with Zeta/PALS particle size and surface potential analyzer (Brookhaven Instruments).
In vitro cytotoxicity
Human breast cancer MCF-7 cells were maintained with DMEM medium containing 10% FBS, 100 U mL−1 penicillin, and 100 μg mL−1 streptomycin at 37 °C in a humidified atmosphere with 5% CO2. The cytotoxicity of magnetic nanoparticles was evaluated by MTT assay. The cells (1 × 104 cells per well) were seeded in 96-well plates and incubated at 37 °C for 24 h. Then the culture medium was replaced with 100 μL of fresh medium containing varied concentration of SPNCs from 0 to 320 μg mL−1 at pH 7.4 and incubated for 24 h. And then the medium was removed and 20 μL of MTT reagent (0.5 mg mL−1 in culture medium) was added. Following incubation for 4 h, 150 μL MTT per medium was removed carefully and dimethyl sulphoxide (DMSO) was added to each well for dissolving the formazan crystals. The absorbance of the solution was measured at 570 nm using a microplate reader (BioTek).
Magnetic resonance imaging (MRI)
MRI studies were performed in a 3.0 T GE Discovery MR750, by using a
sequence (TR = 300 ms, TE = 2.19, 5.10, 8.02, 10.93, 13.84, 16.75, 19.66, and 22.58 ms; ETL: 16 k-space; flip angle = 25 deg; FOV = 120 mm; slice thickness = 2.5 mm; and image size = 256 × 256). The magnetite nanoparticles were dissolved in 2 mL of water with five different concentrations (0, 2, 4, 8, 16 μg mL−1). The corresponding iron concentrations were then determined by inductively coupled plasma (ICP) analysis by using a Perkin-Elmer Optima-5300DV spectrometer (Waltham, Massachusetts, USA). The obtained MR images of FT solutions were used to calculate the r2 values by using a GE AW Volume share 5 program (GE Healthcare/Greater China, Beijing, China).
MSCs culture
Balb/c mouse MSCs and growth medium consisting of basal medium, FBS, glutamine, penicillin, and streptomycin were purchased from Cyagen Biosciences Inc. (Guangzhou, China). MSCs were plated into a 25 cm2 plastic culture flask and were incubated at 37 °C in a 5% CO2 humidified incubator. When the cells were approximately 90% confluence, they were dissociated with 0.25% trypsin–0.04% ethylene diamine tetraacetic acid (EDTA) and passaged. Human breast adenocarcinoma MCF-7 cells were maintained in DMEM medium containing 10% FBS at 37 °C under a 5% CO2 atmosphere.
Prussian blue staining of magnetite nanoparticles labeled MSCs
Approximately 1 × 105 MSCs were seeded in 96-well plate for growth overnight. After incubation with 50 μg mL−1 SPIOs, 85 nm SPNCs or 70 nm SPNCs for the indicated time points, the cells were washed three times with PBS buffer. Subsequently, the cells were stained with prussian blue solution containing 20% (v/v) hydrochloric acid and 10% (v/v) potassium ferrocyanide solution. After incubation for 40 min at room temperature, the cells were washed twice with PBS buffer and were subjected to incubation with a fast red nuclear staining solution for 10 min. Then, the cells were washed twice with PBS and then observed with an Olympus microscope.
Results and discussion
Biocompatible SPNCs were fabricated via a solvothermal method by using sodium citrate as a stabilizer where low content water was introduced to modify the growth of Fe3O4 nanoparticles. Scanning electron microscopy (SEM) images show that the as-obtained SPNCs are all uniform and spherical shape with a rough surface, which is resulted from the primary nanocrystals (Fig. 1 and S1†). It was found that the size of the SPNCs can be well tuned from about 180 to 70 nm by simply increasing the amount of water while keeping all other parameters fixed (Fig. 1 and S1†). It is may be attributed to two reasons. First, the increase of water would accelerate the hydrolysis of the FeCl3 and the formation of more Fe(OH)3, resulting in larger primary Fe3O4 nanocrystals within the SPNCs. It is known that the smaller primary Fe3O4 nanocrystals have higher surface energy, thus they trend to form large aggregated clusters. In contrast, the larger primary nanocrystals would form small aggregated clusters.
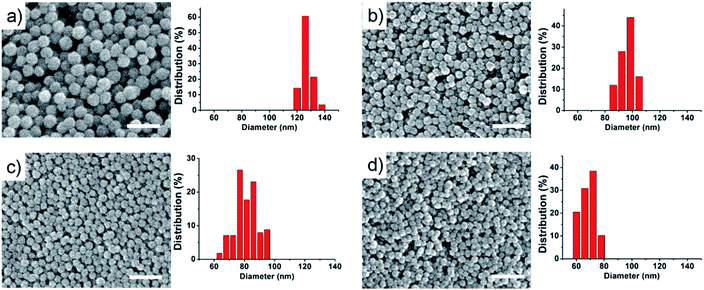 |
| Fig. 1 SEM images and corresponding size distribution plots of colloidal superparamagnetic nanocrystal clusters synthesized by a solvothermal method with different content of water: (a) 1.0, (b) 1.5, (c) 2.0, and (d) 2.5 mL. The average diameters obtained by measuring about 100 particles for each sample, are 130, 100, 85, and 70 nm. All scale bars are 500 nm. | |
Second, water could enhance the electrostatic repulsion of citrate groups on the surface of Fe3O4 nanocrystals, which makes these primary nanocrystals aggregate into small particles to minimize the surface energy. The resultant particle size is much smaller than previous reported results based on solvothermal strategy.34,36
Transmission electron microscopy (TEM) images further reveal that the as-prepared SPNCs have nearly spherical shape and uniform size of 130, 100, 85, and 70 nm, which are well consistent with the SEM observations (Fig. 2a and S2†). Further increased the amount of water to 4.0 mL, the resultant particles would be irregular (Fig. S3†). When pure water as the solvent, uniform α-Fe2O3 nanoparticles would be obtained (Fig. S4†). A magnified TEM image of 85 nm SPNCs shows that the obtained particles are composed of small primary nanocrystals (Fig. 2b). High-resolution (HRTEM) image discloses that the primary nanocrystals are highly crystallized with a size of 4–10 nm and exhibit partially oriented aggregation (Fig. 2c). The distance between two adjacent planes in a specific direction is about 0.25 nm, which can be well assigned to the lattice spacing of (311) planes of Fe3O4. Selected-area electron diffraction (SAED) pattern (Fig. 2d) of a single 85 nm SPNC reveals a polycrystalline nature, further confirming that the particles consist of numerous primary nanocrystals. HRTEM images of the 70 nm SPNCs (Fig. S4 and S5†) show much better crystallographical alignment of primary nanocrystals with slightly larger grains of about 12 nm compared with 85 nm SPNCs, further confirming that water could promote the growth of the Fe3O4 nanocrystals.
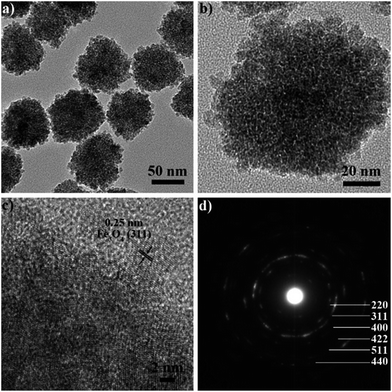 |
| Fig. 2 TEM (a and b) and HRTEM images (c) and selected-area electron diffraction (SAED) pattern (d) of 85 nm colloidal superparamagnetic nanocrystal clusters. | |
X-ray diffraction (XRD) measurement was used to characterize the structure of the obtained SPNCs. The XRD patterns (Fig. 3) reveal six well-resolved diffraction peaks, all of which can be well indexed to the (220), (311), (400), (422), (511) and (440) planes of the cubic Fe3O4 phase (JCPDS no. 19-629). Also, the broad diffraction peaks suggests the nanocrystalline structure of the SPNCs. The average grain sizes of the 130, 100, 85, and 70 nm SPNCs, calculated with the Debye–Scherrer formula for the strongest 311 facet, are 8.5, 10.4, 10.8, and 11.7, respectively. It indicates that the primary Fe3O4 nanocrystals slightly grow large with the increasing of H2O concentration, further confirming that water play a key role in the growth of the magnetite grain.
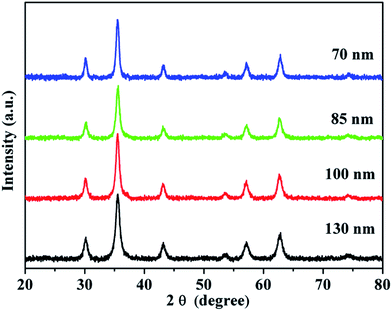 |
| Fig. 3 XRD patterns of 130, 100, 85, and 70 nm colloidal superparamagnetic nanocrystal clusters. | |
The magnetic properties of the obtained SPNCs were investigated using a superconducting quantum interference device (SQUID) magnetometer at 300 K, as shown in Fig. 4. It is observed that the remanence or coercivity of the obtained SPNCs is negligible, reflecting a superparamagnetic property, which can be ascribed to the fact that the grain sizes of the primary nanocrystals are very small (<12 nm). The magnetic saturation values of the 70, 85, 100 and 130 nm SPNCs are measured to be as high as 58.2, 60.7, 63.7, and 66.6 emu g−1, respectively, which rise with the size of particles, indicating that assembly of individual small SPIO into large particle could greatly enhance the response to external field. Furthermore, the FT-IR analysis was used to confirm citrate absorption on the surface, as shown in Fig. S7.† Three characteristic bands at 1637, 1556, and 1420 cm−1 are due to asymmetric and symmetric stretching of COO− on the particle surface, which proves that citrate is absorbed onto the surface of the clusters. The zeta potentials of the 180, 130, 100, 85, and 70 nm SPNCs were measured to be −10.6, −19.3, −21.5, −29.4 and −11.3 mV (Fig. S8†). Then we found that the obtained SPNCs can be well dispersed in various biological media such as H2O, phosphate buffered solution (PBS), Dulbecco's modified eagle medium (DMEM) and fetal bovine serum (FBS) without additional surface modification (Fig. S9†), which indicates that the stabilizer of citrate endows the SPNCs excellent colloidal stability.
 |
| Fig. 4 Magnetic hysteresis loops at 300 K of 130, 100, 85 and 70 nm colloidal superparamagnetic nanocrystal clusters. | |
The superparamagnetic behavior, high magnetization and excellent colloidal stability of the as-prepared SPNCs drove us to investigate their potential in biomedical applications. To measure the transverse relaxation, the in vitro T2-weighted MRI experiments were conducted in agar phantom at 3.0 T. It is observed that the MR signals significantly decrease with the concentration of 70 and 85 nm SPNCs (Fig. 5a). As shown in Fig. 5b, there is a good linear relationship between the r2 values and iron ion concentration. The r2 values are linearly increased along with the iron concentrations. From the slopes of the curves, the r2 relaxivity are calculated up to 102.8 and 175.4 mM−1 s−1 for 70 and 85 nm SPNCs, respectively. The r2 relaxivity of 85 nm SPNCs are markedly high, which make them be a very promising sensitive MRI contrast agent.
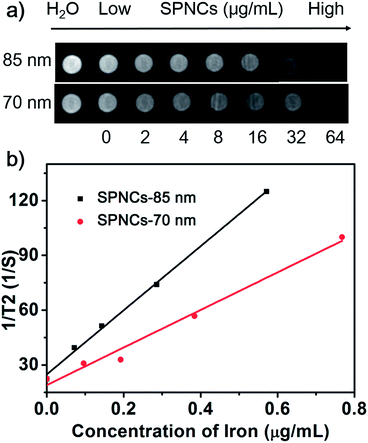 |
| Fig. 5 (a) T2-weighted MRI of phantoms containing 85 and 70 nm colloidal superparamagnetic nanocrystal clusters, (b) dependency of the relaxivity of 85 and 70 nm colloidal superparamagnetic nanocrystal clusters upon iron concentration. | |
To examine the feasibility of the as-obtained SPNCs in biomedical applications, the cytotoxicity was investigated. The effect of the SPNCs (85 and 70 nm) on cell proliferation was assessed with human breast cancer cells (MCF-7 cells) by MTT assay (Fig. S10†). The viability of cells without treatments was assumed to be 100%. Notably, the cell viability still remains above 90% when they were incubated with 20 and 40 μg mL−1 SPNCs. Upon incubating the MCF-7 cells with a high concentration of SPNCs (80 and 160 μg mL−1), less than 15% of the cells died after a 24 h exposure. Even when the concentration was increased to 320 μg mL−1, the cell viability still remains above 80%. These results clearly suggest that both 85 and 70 nm SPNCs have low cytotoxicity due to the presence of biocompatible citrate groups on the surface.
The prominent biocompatibility of the SPNCs motivated us to further investigate their bio-application. SPNCs have been used to label mesenchymal stem cells (MSCs), which can be monitored in vivo by using MRI. To study the uptake of SPNCs, MSCs were incubated with 50 μg mL−1 SPNCs with different time. Iron inside MSC cells can be visualized by prussian blue staining (Fig. 6). After incubation for 4 h, small amounts of SPNCs were uptaken by MSCs. The uptake of SPNCs was obviously increased in all the groups with the incubation time prolonged, indicating that the internalization is time-dependent. After incubation for 24 h, nearly 100% of cells were labeled by 85 and 70 nm SPNCs (Fig. 6). Compared with commercial SPIOs, the intensities of prussian blue staining of cells incubated with either 85 or 70 nm SPNCs are much stronger even incubated for 4 h, indicating that the label efficacy of SPNCs are steady higher than commercial SPIOs.
 |
| Fig. 6 Mesenchymal stem cells (MSCs) labeling with commercial SPIOs, 85 and 70 nm colloidal superparamagnetic nanocrystal clusters within 4, 8 and 24 h. All scale bars are 20 μm. | |
Conclusions
In summary, we have reported a simple recipe for the preparation of sub-100 nm biocompatible SPNCs. The particle size can be facially tuned over a range of 70–180 nm by changing the content of H2O in the system. The as-obtained SPNCs consist of small primary nanocrystals, as a result, which show superparamagnetic properties and high magnetization values. The presence of citrate groups on the surface of SPNCs renders them excellent colloidal stability and good biocompatibility. The resultant 85 nm SPNCs exhibit an ultra-high r2 relaxivity of 175.4 mM−1 s−1, which is promising for MRI. The uptake of SPNCs in MSCs is much higher than that of commercial SPIOs upon the incubation time, which indicate that SPNCs have a high efficacy in labeling MSCs. We believe that the superparamagnetic behavior, high magnetization, high water dispersibility and good biocompatibility of the as-prepared sub-100 nm SPNCs make them ideal candidates for various important applications such as drug delivery, bioseparation, and bioimaging.
Acknowledgements
We greatly appreciate financial support from the National Key Basic Research Program of the PRC (2014CB744504 and 2014CB744501), the National Natural Science Foundation of China (81530054, 81201175, 81371611, 81501537 and 81401469), the Natural Science Foundation of Jiangsu Province (BK20130863), the National Science Foundation for Post-doctoral Scientists of China (2013T60939, 2012M521934, 2013M542576, and 2014T71012), Jiangsu Planned Projects for Postdoctoral Research Funds (1301023A) and the Research Program of Jinling Hospital (2014054).
Notes and references
- Q. Jia, J. Zeng, R. Qiao, L. Jing, L. Peng, F. Gu and M. Gao, J. Am. Chem. Soc., 2011, 133, 19512–19523 CrossRef CAS PubMed.
- C. He, S. Jiang, H. Jin, S. Chen, G. Lin, H. Yao, X. Wang, P. Mi, Z. Ji, Y. Lin, Z. Lin and G. Liu, Biomaterials, 2016, 83, 102–114 CrossRef CAS PubMed.
- S. Inturi, G. Wang, F. Chen, N. K. Banda, V. M. Holers, L. Wu, S. M. Moghimi and D. Simberg, ACS Nano, 2015, 9, 10758–10768 CrossRef CAS PubMed.
- Y. Xu, C. Wu, W. Zhu, C. Xia, D. Wang, H. Zhang, J. Wu, G. Lin, B. Wu, Q. Gong, B. Song and H. Ai, Biomaterials, 2015, 58, 63–71 CrossRef CAS PubMed.
- J.-H. Fang, T.-L. Chiu, W.-C. Huang, Y.-H. Lai, S.-H. Hu, Y.-Y. Chen and S.-Y. Chen, Adv. Healthcare Mater., 2016, 5, 688–695 CrossRef CAS PubMed.
- J. Liu, B. Wang, S. B. Hartono, T. Liu, P. Kantharidis, A. P. Middelberg, G. Q. Lu, L. He and S. Z. Qiao, Biomaterials, 2012, 33, 970–978 CrossRef CAS PubMed.
- J. Zeng, L. Jing, Y. Hou, M. Jiao, R. Qiao, Q. Jia, C. Liu, F. Fang, H. Lei and M. Gao, Adv. Mater., 2014, 26, 2694–2698 CrossRef CAS PubMed.
- R. Qiao, Q. Jia, S. Huwel, R. Xia, T. Liu, F. Gao, H. J. Galla and M. Gao, ACS Nano, 2012, 6, 3304–3310 CrossRef CAS PubMed.
- Z. Zhou, H. Liu, X. Chi, J. Chen, L. Wang, C. Sun, Z. Chen and J. Gao, ACS Appl. Mater. Interfaces, 2015, 7, 28286–28293 Search PubMed.
- J. Zhou, C. Leuschner, C. Kumar, J. F. Hormes and W. O. Soboyejo, Biomaterials, 2006, 27, 2001–2008 CrossRef CAS PubMed.
- J. Estelrich, M. J. Sanchez-Martin and M. A. Busquets, Int. J. Nanomed., 2015, 10, 1727–1741 CAS.
- K. Motomura, T. Izumi, S. Tateishi, Y. Tamaki, Y. Ito, T. Horinouchi and K. Nakanishi, Br. J. Surg., 2016, 103, 60–69 CrossRef CAS PubMed.
- Z. Zhou, C. Wu, H. Liu, X. Zhu, Z. Zhao, L. Wang, Y. Xu, H. Ai and J. Gao, ACS Nano, 2015, 9, 3012–3022 CrossRef CAS PubMed.
- Y. W. Jun, J. W. Seo and J. Cheon, Acc. Chem. Res., 2008, 41, 179–189 CrossRef CAS PubMed.
- M. G. Harisinghani, J. Barentsz, P. F. Hahn, W. M. Deserno, S. Tabatabaei, C. H. van de Kaa, J. de la Rosette and R. Weissleder, N. Engl. J. Med., 2003, 348, 2491–2499 CrossRef PubMed.
- Y. Lv, G. Ding, J. Zhai, Y. Guo, G. Nie and L. Xu, Colloids Surf., B, 2013, 110, 411–418 CrossRef CAS PubMed.
- S. Sharifi, H. Seyednejad, S. Laurent, F. Atyabi, A. A. Saei and M. Mahmoudi, Contrast Media Mol. Imaging, 2015, 10, 329–355 CrossRef CAS PubMed.
- L. Zhu, D. Wang, X. Wei, X. Zhu, J. Li, C. Tu, Y. Su, J. Wu, B. Zhu and D. Yan, J. Controlled Release, 2013, 169, 228–238 CrossRef CAS PubMed.
- C. A. Quinto, P. Mohindra, S. Tong and G. Bao, Nanoscale, 2015, 7, 12728–12736 RSC.
- X. Wan, Y. Song, N. Song, J. Li, L. Yang, Y. Li and H. Tan, Carbohydr. Res., 2016, 419, 33–40 CrossRef CAS PubMed.
- J. Tan, C. Sun, K. Xu, C. Wang and J. Guo, Small, 2015, 11, 6338–6346 CrossRef CAS PubMed.
- D. Sakellari, K. Brintakis, A. Kostopoulou, E. Myrovali, K. Simeonidis, A. Lappas and M. Angelakeris, Mater. Sci. Eng., C, 2016, 58, 187–193 CrossRef CAS PubMed.
- M. Wu, D. Zhang, Y. Zeng, L. Wu, X. Liu and J. Liu, Nanotechnology, 2015, 26, 115102 CrossRef PubMed.
- J. Tan, J. Wan, J. Guo and C. Wang, Chem. Commun., 2015, 51, 17394–17397 RSC.
- G. L. Zhang, J. L. Gao, J. C. Qian, D. Q. Cai, K. Zheng, Z. W. Yu, J. F. Wang, K. Zhong, X. Zhang and Z. Y. Wu, Part. Part. Syst. Charact., 2014, 31, 976–984 CrossRef CAS.
- C. Paquet, H. W. de Haan, D. M. Leek, H. Y. Lin, B. Xiang, G. Tian, A. Kell and B. Simard, ACS Nano, 2011, 5, 3104–3112 CrossRef CAS PubMed.
- C. Paquet, L. Page, A. Kell and B. Simard, Langmuir, 2010, 26, 5388–5396 CrossRef CAS PubMed.
- X. Zhang, X. Xu, T. Li, M. Lin, X. Lin, H. Zhang, H. Sun and B. Yang, ACS Appl. Mater. Interfaces, 2014, 6, 14552–14561 Search PubMed.
- J. C. Li, X. Y. Shi and M. W. Shen, Part. Part. Syst. Charact., 2014, 31, 1223–1237 CrossRef CAS.
- J. Guo, W. L. Yang and C. C. Wang, Adv. Mater., 2013, 25, 5196–5214 CrossRef CAS PubMed.
- S. Laurent, D. Forge, M. Port, A. Roch, C. Robic, L. Vander Elst and R. N. Muller, Chem. Rev., 2008, 108, 2064–2110 CrossRef CAS PubMed.
- T. Hyeon, S. S. Lee, J. Park, Y. Chang and H. B. Na, J. Am. Chem. Soc., 2001, 123, 12798–12801 CrossRef CAS PubMed.
- L. Y. Wang, J. Bao, L. Wang, F. Zhang and Y. D. Li, Chem.–Eur. J., 2006, 12, 6341–6347 CrossRef CAS PubMed.
- J. Park, K. An, Y. Hwang, J. G. Park, H. J. Noh, J. Y. Kim, J. H. Park, N. M. Hwang and T. Hyeon, Nat. Mater., 2004, 3, 891–895 CrossRef CAS PubMed.
- Y. W. Tan, Z. B. Zhuang, Q. Peng and Y. D. Li, Chem. Mater., 2008, 20, 5029–5034 CrossRef CAS.
- R. Fu, X. Jin, J. Liang, W. Zheng, J. Zhuang and W. Yang, J. Mater. Chem., 2011, 21, 4 Search PubMed.
- H. Deng, X. L. Li, Q. Peng, X. Wang, J. P. Chen and Y. D. Li, Angew. Chem., Int. Ed., 2005, 44, 2782–2785 CrossRef CAS PubMed.
- J. Ge, Y. Hu, M. Biasini, W. P. Beyermann and Y. Yin, Angew. Chem., Int. Ed., 2007, 46, 4342–4345 CrossRef CAS PubMed.
- J. Liu, Z. Sun, Y. Deng, Y. Zou, C. Li, X. Guo, L. Xiong, Y. Gao, F. Li and D. Zhao, Angew. Chem., Int. Ed., 2009, 48, 5875–5879 CrossRef CAS PubMed.
- F. Roohi, J. Lohrke, A. Ide, G. Schutz and K. Dassler, Int. J. Nanomed., 2012, 7, 4447–4458 CAS.
- Z. Popovic, W. Liu, V. P. Chauhan, J. Lee, C. Wong, A. B. Greytak, N. Insin, D. G. Nocera, D. Fukumura, R. K. Jain and M. G. Bawendi, Angew. Chem., Int. Ed., 2010, 49, 8649–8652 CrossRef CAS PubMed.
Footnote |
† Electronic supplementary information (ESI) available. See DOI: 10.1039/c6ra09344b |
|
This journal is © The Royal Society of Chemistry 2016 |
Click here to see how this site uses Cookies. View our privacy policy here.