DOI:
10.1039/C6RA11695G
(Review Article)
RSC Adv., 2016,
6, 60277-60286
Advances in biogenic synthesis of palladium nanoparticles
Received
5th May 2016
, Accepted 11th June 2016
First published on 14th June 2016
Abstract
Green approaches for the synthesis of nanoparticles provide advantages due to the fact that green protocols are benign and environmentally friendly. Among various green recipes, biogenic synthesis of nanoparticles has recently emerged as an active area of research due to the simplicity of this method, with cost effective protocols, higher potential of reduction and low toxic effect on human health and the environment. Moreover, the biogenic reduction occurs at physiological conditions of temperature and pressure. The raw materials are easily available and therefore, the reaction can easily be scaled up. This paper presents a review to give an idea about the most reliable, cost-effective and environment friendly synthetic protocols for metal nanoparticles to control size, shape and dispersity. In this contribution, we have emphasized particularly various plants responsible, and investigated so far, for the synthesis of palladium nanoparticles. Based on the isolated compounds/metabolites such as polyols, polyphenols, flavonoids and proteins from the respective plant extracts, a correlation is established where such metabolites are linked with the biogenic syntheses of Pd nanoparticles. The present investigation and works reviewed lead us to recommend a few potential plants for the reduction of Pd2+ ions into Pd0 nanoparticles. This review not only summarizes the present literature but also highlights the potential of this field to open up new avenues for researchers.
1 Introduction
In the last couple of years, the biogenic synthesis of nanomaterials has attracted the attention of nanotechnologists and material scientists, due to the growing environmental contamination caused by conventional chemical methods.1–5 Depending on the availability and feasibility of instrumentation and required chemicals, there is a plethora of methods (both chemical and physical) already established in the scientific community to synthesize nanomaterials to achieve specific applications.6–10 However, in the case of physical processes, certain essential requirements have to be fulfilled that may include, maintaining high temperature and pressure, which require a lot of energy.11,12 Additionally, in the chemical methods, one requires reagents such as reducing agents, starting materials and solvents which are not only very much costly but also hazardous and toxic to the environment.2,13 Moreover, chemical synthesis results in adsorbing or binding the chemical surfactants or toxic molecules to surfaces of nanomaterials which prevent their use in many applications, especially in biomedical sciences. A recent review demonstrates earlier works on the toxic effect of silver nanoparticles (NPs) in living systems.14 Therefore, there is an ever-growing need to develop and promote the biogenic synthesis of nanomaterials which are more environmentally friendly and sustainable to meet the future requirements of technology.
There are many reports on the synthesis of NPs through biological systems utilizing bacteria, fungi15,16 and plants.17,18 Although, these methods are also eco-friendly and easy to use but present review is restricted only to the plant mediated synthesis of palladium NPs (PdNPs) while synthesis of such NPs through other biological systems will be discussed only very briefly. For example, microbes also offer biogenic synthesis of metallic NPs. However, in addition to the requirement of skilled professionals and harsh experimental conditions, slow synthesis process and chances of contamination and infection are the challenges to be addressed in such synthetic protocols.19,20
Literature show that various NPs such as gold, silver, palladium, platinum etc. have been synthesized using plant extracts.21,22 Different parts of plant like stem, latex, and roots are being exploited for the synthesis of metal NPs. The reduction properties of plant parts are attributed to the presence of various phytochemicals such as polyphenols, alkaloids and terpenoids. Although, extensive published data on the biogenic synthesis of gold and silver NPs can be found, very few studies are reported on the biogenic synthesis of PdNPs. PdNPs have wide range of applications in different areas, for example as catalysts for various chemical transformation,23,24 carbon–carbon bond formation,25 in fuel cell electrochemical reactions,26 hydrogenation reactions,27 Suzuki coupling reactions,28 Suzuki–Miyaura and Mizoroki–Heck reactions29,30 and in Stille and Sonogashira coupling reactions.31,32 Therefore, developing environmentally friendly methods for the synthesis of PdNPs is of high value. The foremost purpose of the present contribution is to highlight the most reliable, cost-effective and environment friendly synthetic protocols for metal NPs of different size, shape, composition, and with a high degree of monodispersity. More precisely, this review highlights biogenic synthesis of PdNPs with a particular emphasis on the formation mechanism and factors affecting size, morphology and crystallinity. In addition, we have reviewed the literature on the green synthesis of PdNPs using molecular bio-reductants and plant metabolites from the extracts investigated for the synthesis of NPs, especially involving reduction of Pd2+ ions are briefly discussed. Such information lead us to recommend few potential plants for the synthesis of PdNPs.
2 Methods for the synthesis of palladium nanoparticles
2.1 Chemical and physical methods
Various chemical and physical methods have been exploited for the synthesis of PdNPs. For example, Nguyen et al.33 synthesized PdNPs by reducing Na2PdCl4 using ethylene glycol (EG) as reducing agent and polyvinylpyrrolidone (PVP) as stabilizer. Similarly, Bonet et al.,34 synthesized monodisperse PdNPs using EG and PVP. Shape and size of PdNPs were controlled by varying the temperature, initial concentration of metal precursor and amount of stabilizing ligand i.e. PVP. A recent review summarizes various works on the chemical synthesis of silver NPs through microemulsion technique and also various parameters influencing the formation of similar particles.35 Similarly, another review summarizes the toxicity and risks associated with various nanomaterials.36 Since review of works related to chemical and physical methods for the synthesis of NPs, especially PdNPs, is not the aim of the present contribution, we emphasize more on the adverse impacts of such methods. Therefore, green methods for the synthesis of NPs are advantageous in comparison to chemical and physical methods because such methods prevent the production of toxic waste, cost effective scaled up synthesis, high yield, do not require toxic reducing agents and solvents, process can be carried out at room temperature and ambient pressure. Furthermore, renewable materials are used as precursors for the synthesis, biodegradable materials as stabilizing/capping agents and last but not the least green methods avoid the use of sophisticated and complex equipment leading to much safer chemistry.
2.2 Microbial methods
Green synthesis of PdNPs using microbes has been reported37 where Geobacter sulfurreducens, (anaerobic, Gram negative bacteria) were used for the synthesis of PdNPs. Additionally, Escherichia coli mutant strains MC4100, IC009, IC010, FTD89 and FTD150 were also used to reduce Pd2+ salt to Pd0.38 Two model organisms such as sulfate reducing bacterium Desulfovibrio desulfuricans, and metal respiring bacterium Shewanella oneidensis were used for the synthesis of Pd0 NPs.39,40 However, in most of the cases, requirement of huge amounts of hydrogen which acts as an electron donor is considered as major and inherent drawback making the process economically less viable.41 In another study, vitamin B1 (present in bacteria and fungi) was also used as potential reducing agent for the synthesis of PdNPs where nano-belts, nano-plates and nano-trees of Pd were synthesized. The synthesis was carried at room temperature without using any capping agent.42 Since the review of all the microbial methods for the synthesis of metallic NPs is not the scope of present review, brief detail given above are just to give the overview and highlight the use of these bio-reductants for green synthesis of NPs.
2.3 Plants mediated synthesis
Interaction of metal ions and various plants species have traditionally been used to purify ground water and to cope with soil salinity.43 There are different in-built cellular mechanisms including phytoremediation, phytostabilization, phytoaccumulation to detoxify the metals in plants44 and on this ground plants are highly specific in their functions.45 Also in some of the recent studies, metals as small NPs, were found in plants by reduction of the metal ions absorbed as soluble salts.46–48 In fact the structure function relationship of plant with metals and environment has led the basis for the development of a plant-mediated synthesis of metal NPs.
Recent literature show that plants are extensively exploited for the synthesis of various NPs where metal salt solution is mixed with plant extract at room temperature or at elevated temperature and stirred to reduce the metal leading nucleation and growth of respective metal NPs. In such synthetic protocols plants extracts not only act as reducing agents but also as stabilizing agent while the size and morphology of NPs is affected by the concentration of plants extract, concentration of metals salt, pH, temperature and reaction time.49–51 Various plants and their parts have been exploited for the synthesis of silver, gold and palladium NPs. Among parts of plants, leaves, fruits, roots have extensively been used for the synthesis of NPs.
2.3.1 Leaf extract mediated synthesis of PdNPs. Among various parts of plants, leaves have proven to be the best raw material to synthesize metal nanoparticles. It could be due to the fact that plants leaves are the chemical factories of plants where most of the electrochemical reactions take place. Leaf extract of Anacardium occidentale has been reported for the synthesis of PdNPs where particle size of 2.5 to 4.5 nm was achieved while the particles were found to be spherical in morphology.52 It was proposed and spectroscopically (FTIR) proven that polyols present in the extract of Anacardium occidentale were responsible for the reduction of Pd2+ ions, forming NPs which were further stabilized by proteins and negatively charged carboxylate ions (Fig. 1).
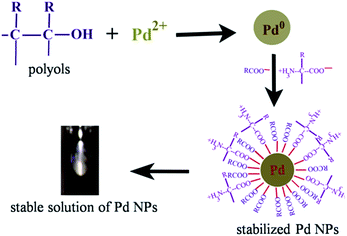 |
| Fig. 1 Possible mechanism for the synthesis/stable solution of PdNPs using leaf extract of Anacardium occidentale. Reproduced with permission from ref. 52. | |
In another report, Cinnamomum camphora leaves were used for the biogenic synthesis of PdNPs where particles obtained were in the size range of 3.2 to 6.0 nm.53 In this investigation, it was demonstrated through FTIR analysis that polyols namely flavones, terpenoids, polysaccharides and water-soluble heterocyclic compounds present in the extract of plant are responsible for the reduction of PdCl2 salt. In another study, leaf extract of Soybean (Glycine max) was used to synthesize PdNPs with an average size of 15 nm.54 Since leaf extract of Soybean, as characterized using FTIR analysis, were found to contain large amounts of proteins, enzymes and some aminoacids, these constituents were assumed to be responsible for the reduction of Pd2+ ions. In another study, authors exploited the leaf extract of Artemisia annua to synthesize PdNPs using solution of PdCl2 (50 ml of 1.7 × 10−4 M) as Pd precursor where the particles were found to show size range between 20 and 30 nm.55 In this experiment, reduction process was completed within a short time, as indicated by the change in color from light to dark brown. It was again demonstrated through FTIR analysis that chemical constituents such as polyols and compounds with carbonyl groups are responsible for the reduction process (Fig. 2). Authors proposed that polyols and compounds with carbonyl groups present in the aqueous extract of plant, bridge the Pd2+ ions, forming a metal ion complex which in turn reduce the palladium ions to Pd0 at the expense of bound organic moieties which normally get oxidized. The reduced Pd0 atoms undergo agglomeration/clustering which act as nucleation centers leading to growth process to form NPs. The phytomolecules act both as reductants and stabilizers. Therefore, the size and morphology of such NPs can easily be controlled by changing the ratio between the concentration of plant extract and the metal salt.
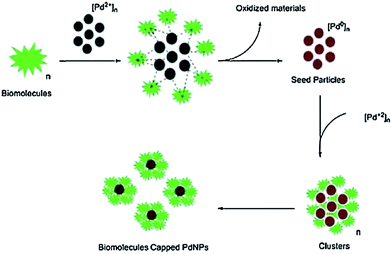 |
| Fig. 2 Possible mechanism for the reduction of Pd2+ ions using A. annua leaf extract. Reproduced with permission from ref. 55. | |
Similarly, a recent study showed the use of Catharanthus roseus leaf extract as reducing agent for the synthesis of PdNPs with an average size of 38 nm.56 Since it was again demonstrated through FTIR analysis that secondary metabolites such as phenolic compounds are present in the leaf extract of C. roseus, such metabolites were assumed to reduce Pd2+ ions. In this experiment, palladium salt used was palladium acetate [Pd(OAc)2] and the experiment was carried out at 60 °C (Fig. 3).
 |
| Fig. 3 Proposed mechanism of the reduction of Pd2+ ions by the extract of C. roseus. Reproduced with permission from ref. 56. | |
Similarly, another report demonstrated the use of leaf extract of Delonix regia for the synthesis of PdNPs with an average size of 19 nm. The as synthesized NPs were polycrystalline with crystallite size ranging from 2 to 4 nm.57 Authors further reported that water-soluble phenolic acid (gallic acid) and flavonoid compounds present (analyzed using FTIR) could be responsible for the reduction of Pd2+ ions (Fig. 4).
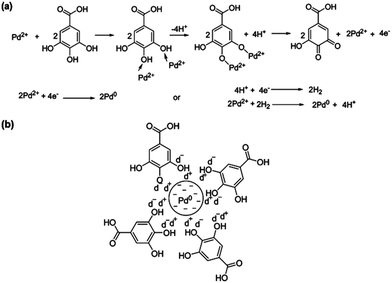 |
| Fig. 4 Probable bio-reduction mechanism of Pd2+ to Pd0 (a) bio-reduction and (b) stabilization of PdNPs using gallic acid. Reproduced with permission from ref. 57. | |
Fig. 4 shows the proposed mechanism for the bio-reduction of Pd2+ ions using gallic acid where hydroxyl groups present on the benzene ring of gallic acid are assumed to donate electron to reduce Pd2+ ions. Since phenolic compounds have the capability of donating electrons, forming quinones, it has been assumed that the oxidation of gallic acid by PdCl2 results in the formation of intermediate Pd complex followed by the conversion of gallic acid into its quinone form and reduction of PdCl2 to Pd0.
Similarly, essential oils extracted from the leaves of medicinal plants such as Coleus aromaticus and Myristica fragrans were used for the synthesis of PdNPs where the particle size achieved was ∼2.8 nm.58 The authors of the study demonstrated through FTIR analysis that terpenoids and phenolic ether derivatives present in the extracts participated in the reduction process as well as acted as capping agents to stabilize the formed NPs. The synthesized PdNPs exhibited high catalytic activity in the degradation of organic pollutants such as methyl red, methyl orange, Eriochrome black T, methylene blue, rhodamine B, ortho-, meta- and para-nitrophenols. Another study shows the reduction of PdCl2 ions to PdNPs in the presence of Euphorbia granulate plant extract.59 The reaction between plant extract and PdCl2 salt was carried out for 5 minutes at 80 °C where reduction was again found to be attributed to the presence of relatively high contents of flavonoids and phenolic acids, demonstrated through FTIR analysis.
In another study, leaf extract of Hippophae rhamnoides Linn were used for the synthesis of PdNPs where the reduction of PdCl2 salt using plant extract was carried out at 80 °C and color of the solution was changed from transparent yellow to dark brown in about 25 minutes. It was again demonstrated through FTIR analysis that flavonoids and phenolic compounds present in the extract were actually responsible for the reduction of PdCl2 salt as well as stabilization of resulted PdNPs.60 Similarly, in another study, Piper betle leaf broth extract was exploited for the synthesis of PdNPs.61 In this case, NPs synthesized were found to show spherical shape, with a very narrow size distribution and with an average particle size of 4 ± 1 nm. The FTIR studies showed that flavonoids and proteins present in the extract were responsible for the reduction and stabilization process.
In another detailed study, authors employed Solanum trilobatum leaf extract to photo-synthesized PdNPs under moderate pH (pH 7) and at room temperature.62 In this process conjugated lipoic acid (S-PdNp–LA) and vitamins (S-PdNp–Vitamin–LA) were exploited for the stabilization process. These PdNPs were found to be of various morphologies with size ranging from 60 to 70 nm (S-PdNp), 65 to 80 nm (S-PdNp–LA) and 75 to 100 nm (S-PdNp–Vitamin–LA). Since the extract of Solanum trilobatum contains water soluble compounds such as proteins, polyols, flavones, terpenoids characterized using FTIR spectroscopy, such constituents actually promoted the reduction process. Similarly, in another study, leaf extract of Andean blackberry was ultrasonicated and further used for the synthesis of PdNPs.63 NPs obtained through this process were crystalline in nature with decahedron morphology and size around 55–60 nm. These NPs were further used as photocatalyst for the decomposition of methylene blue (MB) using sunlight and an enhanced photocatalytic activity (>72%, k = 0.0021614 min−1) for PdNPs was observed due to electron relay effect. This work shows great promise for biogenic synthesis of NPs for various applications where detailed investigation is desired.
List of various plants exploited for the synthesis of PdNPs is shown in Table 1. In most of the cases, leaves extracts of the respective plants are exploited for the synthesis of PdNPs. The data further show the type of constituents isolated from the extracts where it can be assumed that such constituents play major role in the reduction as well as capping/stabilization of NPs. Such a comparison can lead to exploit other plant extracts with similar constituents for the synthesis of various metal NPs, especially industrially important PdNPs.
Table 1 Leaves extracts of various plants used for the synthesis of PdNPs
Plant |
Part used |
Size and shape of NPs |
Biomolecules involved |
Reference |
Anacardium occidentale |
Leaves |
2.5–4.5 nm, spherical |
Polyols and proteins |
52 |
Andean blackberry |
Leaves |
55–60 nm, decahedron |
Flavonoids, ellagic acid, tannins |
63 |
Artemisia annua |
Leaves |
20 to 30 nm, spherical |
Polyols |
55 |
Catharanthus roseus |
Leaves |
38 nm, spherical |
4-Methylmyoinositol |
56 |
Cinnamomum camphora L. |
Leaves broth |
3.2–6.0 nm, quasi-spherical |
Flavones, terpenoids and polysaccharides |
53 |
Delonix regia |
Leaves |
2–4 nm, shape not given |
Water soluble polyols |
57 |
Euphorbia granulate |
Leaves |
25–35 nm, shape not given |
Flavonoids, phenolic acids |
59 |
Hippophae rhamnoides Linn |
Leaves |
Size not give, spherical |
Flavonoids, phenolic contents |
60 |
Piper betle |
Leaves broth |
4 ± 1 nm, spherical |
Flavonoids, proteins |
61 |
Solanum trilobatum |
Leaves |
60–100 nm, spherical |
Flavones |
62 |
Soybean |
Leaves |
∼15 nm, spherical |
Proteins, amino acids |
54 |
2.3.2 Fruit extracts mediated synthesis of PdNPs. Similarly, fruit extracts of some of the plants have also been reported for the synthesis of PdNPs. A recent report shows the reduction of graphene oxide (GO) and Pd2+ ions by fruit extract of Berberis vulgaris to prepare PdNPs supported on reduced graphene oxide nanocomposites.64 The reaction between fruit extract and PdCl2 was completed in 10 minutes at 80 °C. The used extract was investigated using FTIR which showed the presence of flavonoids, vitamins (vitamin C), phenolic acids and such constituents were supposed to act as both reducing and stabilizing agents for the synthesis of PdNPs. In this reduction process, vitamin C present in the barberry fruit extract was considered to play a major role where –OH functional groups present in the primary structure undergo Red/Ox reaction through transfer of electrons and form metal NPs through metal ions reduction. These –OH functional groups are supposed to be converted to carbonyl groups during Red/Ox reaction.In a pursuit to investigate the effect of phenolic and triterpenoidic groups present in the fruit extract of Pistacia atlantica on the reduction of Pd2+ ions, Molaie et al.,65 carried out a recent study and proposed that such constituents act as reducing as well as stabilizing agent. The average size of the as synthesized NPs was found to be ∼60 nm with spherical morphology. Similarly, aqueous extract of Terminalia chebula was also investigated for the synthesis of iron and PdNPs.66 The as synthesized amorphous iron NPs were found to be less than 80 nm in size while cube shape PdNPs were found to be of less than 100 nm size. It was again demonstrated through FTIR analysis that polyphenols present in the plant extract were actually acting as reducing and capping agents. Similarly, in another study, extract of Vitis vinifera was used for the synthesis of Au, Ag, Pt and PdNPs where PdNPs were found to be of spherical morphology with the size range of 5–10 nm. In this case, again polyphenols, analyzed through FTIR, were considered to be responsible for Pd2+ ions reduction.67 Extract of Gardenia jasminoides Ellis has also been exploited for the synthesis of PdNPs in the range of 3–5 nm.68 The synthesized NPs showed high dispersity at 70 °C while antioxidants compounds such as geniposide, chlorogenic acid, crocins and crocetin, analyzed through FTIR, were assumed to act as reducing and stabilizing agents. In another recent report, Annona squamosa L. peel extract was investigated for the synthesis of PdNPs from the solution of Pd(OAc)2.69 In this case, the reaction was carried out at 60 °C and the as synthesized NPs showed spherical morphology with size ∼80 ± 5 nm. Fig. 5 below depicts the proposed reduction mechanism of Pd2+ ions where it has again been proposed, based on the FTIR analysis, that compounds containing water soluble hydroxy functional groups present in the plant extract initiated the reduction process as well as acted as stabilizing agents. Similarly, in another report, reduction of Pd2+ ions into PdNPs was carried out using the extract of banana peel where again based on FTIR analysis, the reduction process was proposed to be carried out by compounds containing carboxyl, amine and hydroxyl functional groups.70 In this investigation, reaction was completed in only 3 minutes at 80 °C while the size of the synthesized PdNPs was found to be around 50 nm.
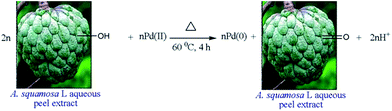 |
| Fig. 5 Proposed mechanism for the reduction of Pd2+ ions from peel extract of A. squamosa L. Reproduced with permission from ref. 69. | |
Table 2 summarizes plants where fruit extract of the respective plant has been exploited and reported for the synthesis of PdNPs. Data also show the type and nature of constituents present in the plants which were assumed to take part in the reduction as well as stabilization of NPs. It indicates that in majority of the cases, compounds with hydroxyl functional groups were found to initiate the reduction process. Such information can further be exploited in selecting so far un-explored plants with similar constituents for the synthesis of Pd and other metallic NPs.
Table 2 Fruit extracts of plants used for the synthesis of PdNPs
Plant |
Part used |
Size/shape of NPs |
Biomolecules involved |
Reference |
Annona squamosa L. |
Fruit peel |
80 ± 5 nm, spherical |
Hydroxyl groups |
69 |
Banana peel (Musa paradisiaca) |
Fruit peel |
50 nm, crystalline and irregular shape |
Carboxyl, amine and hydroxyl groups |
70 |
Berberis vulgaris |
Fruit |
18 nm, spherical |
Phenols, flavonoids, vitamins |
64 |
Gardenia jasminoides Ellis |
Fruit |
3–5 nm, shape not given |
Chlorogenic acid, crocins and crocetin |
68 |
Pistacia atlantica |
Fruit |
60 nm, spherical |
Water-soluble polyols |
65 |
Terminalia chebula |
Fruit |
100 nm, cubic |
Polyphenols |
66 |
Vitis vinifera |
Fruit |
44–50 nm, spherical |
(Not mentioned) |
67 |
2.3.3 Whole plant extracts mediated synthesis of PdNPs. In addition to leaves and fruits, extracts of other parts of plants have also been reported for the synthesis of PdNPs. In a similar attempt, extract of terrestrial weed Antigonon leptopus has been investigated for the synthesis of PdNPs. In this experiment, PdCl2 salt and weed extract were heated at 95 °C for five minutes where dark brown color indicated the formation of PdNPs.71 Furthermore, through FTIR analysis, it has been suggested that flavonoids, phenolic acids and proteins present in the extract were actually responsible for the reduction and stabilization of nuclei and grown NPs. Similarly, another report shows the use of tea and coffee extract for the synthesis of bulk quantities of Ag and PdNPs.72 The reaction was carried out at room temperature while the NPs were found to be spherical in shape with size ranging from 20–60 nm. It was further proposed that polyphenols present in the plant extract actually initiated the reduction process and also acted as capping agents. In an attempt to exploit various parts of plants for the synthesis of NPs, root extract of Asparagus racemosus Linn has been investigated for the synthesis of highly stable Pd and PtNPs.73 Report shows that the produced PdNPs were of spherical morphology and were highly crystalline with particle size in the range from 1 to 6 nm. Phototropins and flavones present in the extract were assumed to act as reducing agents for the synthesis of PdNPs. However, authors did not carry out FTIR or related characterization techniques to confirm the presence of flavones and related compounds. Similarly, production of spherical PdNPs using bark extract of Cinnamon zeylanicum is recently reported by Sathishkumar et al.,74 where size of the synthesized particles was found to be in the range of 15–20 nm. It has further shown that since bark of Cinnamon zeylanicum has polyols such as terpenoids, flavones and polysaccharides, such constituents were actually responsible for the reduction process while proteins present in the extract acted as capping and stabilizing agents. Similarly, another recent report shows the use of hearth wood extract of Artocarpus lakoocha Roxb for the synthesis of PdNPs.75 This report also shows that the cellulose supported PdNPs were found to exhibit enhanced catalytic activity for the Suzuki and Heck coupling reactions in water under microwave heating. Such NPs were found to be of 10–30 nm in size, highly crystalline in nature with spherical morphology and also exhibited excellent thermo and air stability. Fig. 6 below represents biogenic production of PdNPs using hearth wood extract of A. lakoocha Roxb as well as catalytic activity towards Suzuki and Heck coupling reactions.
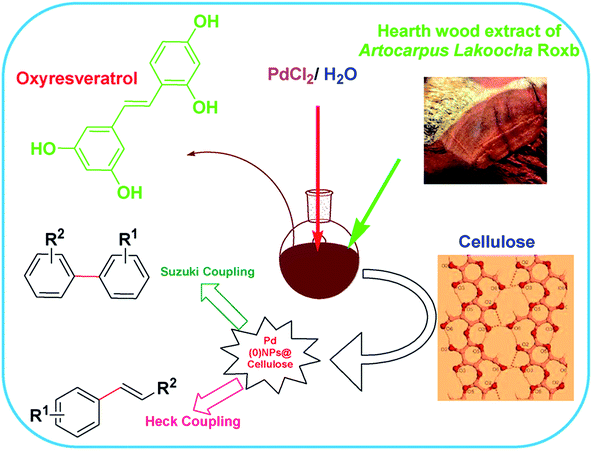 |
| Fig. 6 Schematic representation of the biogenic synthesis of PdNPs and its catalytic activity towards Suzuki and Heck coupling reactions. Reproduced with permission from ref. 75. | |
Similarly, another recent report shows the synthesis of PdNPs employing the extract of Pulicaria glutinosa where the synthesized NPs were investigated to display excellent catalytic activity towards Suzuki coupling reaction.50In this report, the synthesized PdNPs were fully characterized using UV-Vis spectroscopy, X-ray diffraction (XRD), transmission electron microscopy (TEM), energy dispersive X-ray spectroscopy (EDX) and Fourier Transform-Infrared Spectroscopy (FT-IR). Morphology and size of the as-synthesized PdNPs, as per investigated using TEM, revealed PdNPs with particles with diameter of ∼20–25 nm (Fig. 7) where the presence of an organic layer (light contrast) around NPs can also be seen in the magnified image (Fig. 7c). The FFT along with d-spacing measured on HRTEM image showed the face centered cubic phase of as synthesized nanoparticles. It is further proposed based on FT-IR and UV/Vis spectroscopy that compounds with hydroxyl groups present in the plant extract were actually responsible for the reduction process. Analysis of the extract showed the presence of flavonoids and polyphenols in addition to other phytochemicals.
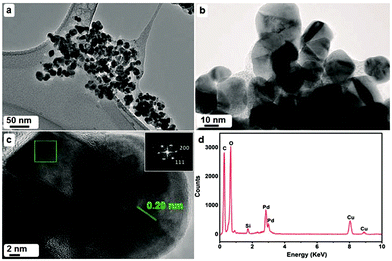 |
| Fig. 7 Transmission electron microscope (TEM) and high resolution (HRTEM) images of the PdNPs (a) overview, (b) magnified HRTEM image, (c) HRTEM image with labelled d-spacing and FFT (as an inset on top right corner) taken from the place marked as green box. (d) Energy dispersive X-ray spectrum (EDX) of as-synthesized PdNPs confirming the composition of product. | |
2.3.4 Plants polymers mediated synthesis of PdNPs. There are number of reports in the literature where natural plants polymers have also been used for the biosynthesis of PdNPs. In one such experiment, gum ghatti (Anogeissus latifolia) has been investigated for the synthesis of PdNPs (4.8 nm size) where proteins, compounds with carboxylic and hydroxyl groups present in gum ghatti were assumed to act as reducing as well as stabilizing agents.76 However, authors did not carry out characterization techniques such as FTIR, NMR etc. to confirm the presence of above compounds. Similarly, another study exploited the use of gum olibanum (a biopolymer) for the reduction of Pd2+ ions into Pd0 NPs.77 These NPs were found to be of spherical in shape, uniform and with average particle size around 6.6 ± 1.5 nm. In this case, compounds with hydroxyl/carboxyl groups and proteins present in the gum were again assumed to be responsible for the reduction and capping process. Another recent report shows the use of Sour cherry tree gum for the synthesis of PdNPs.78 The average size of the synthesized NPs was found to be 5 ± 2 nm and particles were found to be of spherical shape.Table 3 summarizes the plants with their different parts used for the synthesis of PdNPs. It is again evident that in most of the cases, polyphenols and compounds with hydroxyl and carboxylic groups are found to be the major constituents of the extracts and are assumed to initiate the reduction process of Pd salts or other precursors in addition to stabilizing and capping the synthesized NPs. Such information can further be exploited to select plants with same/similar constituents to investigate their biogenic potential, in particular for the synthesis of metallic NPs. Exploiting such/similar plants for the bulk production of NPs is essentially required since such metallic NPs can be used to initiate and catalyze many chemical reactions. A green approach to synthesize and stabilize such metallic NPs for various catalytic reactions is also essential for environmental remediation.
Table 3 List of plants with plant parts used for the synthesis of PdNPs
Plant |
Part used |
Size/shape of NPs |
Biomolecules involved |
Reference |
Anogeissus latifolia |
Gum |
4.8 ± 1.6 nm, spherical |
Hydroxyl, carboxylate groups, proteins |
76 |
Astraglmanna |
Whole plant |
15 nm, spherical |
(Not-mentioned) |
79 |
Asparagus racemosus Linn |
Root |
1–6 nm, spherical |
Phototropins, flavones |
73 |
Artocarpus lakoocha Roxb |
Hearth wood |
10–30 nm, spherical |
Hydroxyl groups |
75 |
Beet juice |
Juice |
5 nm, spherical |
Phenols/methoxy phenols |
80 |
Cinnamomum zeylanicum Blume |
Bark |
15–20 nm, spherical |
Terpenoids |
74 |
Coleus amboinicus |
Whole plant |
10–28 nm, spherical and triangular |
Polyols-flavones, terpenoids, polysaccharides |
81 |
Curcuma longa |
Tuber |
10 to 15 nm, spherical |
Polyols and proteins |
82 |
Gum olibanum |
Gum |
6.6 nm, spherical |
Hydroxyl/carboxylic groups |
77 |
Pulicaria glutinosa |
Whole plant |
20–25 nm, spherical |
Flavonoids and polyphenols |
50 |
Red grapes |
Pomace |
5–10 nm, spherical |
Polyphenols |
67 |
Sargassum ilicifolium |
Whole plant |
60 to 80 nm, spherical |
Proteins, ascorbic acid |
83 |
Tea and coffee extract |
Coffee/tea powder |
20–60 nm, spherical |
Caffeine/polyphenols |
72 |
Watermelon |
Watermelon rind |
96 nm, spherical |
Polyhydroxyl groups |
84 |
2.3.5 Synthesis of bimetallic NPs. Foregoing part was mostly focused on the available research exploiting various plants and their various parts for the synthesis of PdNPs. However, bimetallic NPs have recently been under focus as these NPs offer enhanced activities in comparison to their single domains due to the interfacial electronic communication. Several bimetallic NPs have been synthesized using plant extracts while additional work is essentially required in exploiting the unexplored plants. In this connection, a recent report shows the synthesis of Fe/PdNPs using grapes leaf extract.85 Such NPs have been exploited for environmental remediation and the data indicate that these bimetallic NPs (Fe/Pd) show far better capability in removing orange II in 12 hours in comparison to only Fe NPs. Similarly, a leaf extract of Cacumen platycladi was used to produce Au/PdNPs with 7.4 nm mean size86 and the analysis of this extract through FTIR suggested the presence of water soluble polyhydroxy biomolecules such as flavonoids and reducing sugars which actually took part in the bio-reduction process. Similarly, another report shows the use of the leaf extract of Myrtus communis L. as non-toxic reducing agent for the synthesis of Pd/TiO2NPs.87 This investigation again shows the presence of water soluble heterocyclic compounds such as flavones which were assumed to carry out reduction process. Another recent study shows the use of seed extract of Theobroma cacao L. for the synthesis of Pd/CuONPs88 where flavonols such as epicatechin, catechin and their polymeric derivatives, analyzed through FTIR, were considered to be responsible for the reduction process. In this study, the average size of the synthesized NPs (Pd/CuO) was found to be 40 nm.Recently, several efforts have been made for the synthesis of heterogeneous catalysts and magnetic nanocatalysts exploiting biogenic synthetic protocols. For example, root extract of Euphorbia stracheyi Boiss was used for the synthesis of Pd/Fe3O4NPs.89 The reduction process in this case was attributed to the presence of phenolic compounds in the extract, analyzed through FTIR. The synthesized NPs were found to be of spherical shape with an average size of 10 nm and showed some agglomeration due to their magnetic nature. Similarly, using root extract of Euphorbia condylocarpa M. Bieb, NPs such as Pd/Fe3O4 have been synthesized.90 In this investigation, again it was demonstrated through FTIR analysis that flavonoids and phenolic acids present in the plant extract were responsible for the reduction process. The synthesized NPs were of less than 39 nm size and were found to be magnetically recoverable catalyst for phosphine free Sonogashira and Suzuki coupling reactions.
Analysis of data on the constituents of various plants presented above (Tables 1–4) indicated the presence of flavonoids, polyphenols and water soluble polyols as well as proteins in most of the extracts whether obtained from the whole plant or plant parts. Additionally, such constituents were assumed to act as reducing agents (reduction of PdCl2 salt) for the synthesis of PdNPs while proteins acted as stabilizing ligand. Such constituents can be found in many other plants which are not investigated so far for the biogenic synthesis of PdNPs. In a report fruit extract of Malus domestica has been exploited for the synthesis of silver NPs where the extract is found to contains proteins, flavonoids, polyols and reducing sugars.92 Such plant extracts show potential for the biogenic synthesis of PdNPs and should be investigated. Similarly, Rosa damascenea is also rich in flavonoids, polyphenolic compounds and essential oils which are essential components for the reduction of Pd salt.93 The extract has been exploited for the synthesis of Ag and AuNPs but not for the PdNPs and hence shows potential to be investigated for the synthesis of PdNPs as well.94 Similarly, juice of Punica granatum has been found to be rich in different organic compounds such as organic acids, amino acids, sterols, terpenoids, fatty acids, hydroxybenzoic acids, flavones, anthocyanines, etc.95 Compounds such as ellagic acid, gallic acid, quinic acid, caffeic acid, catechin, (−)epicatechin, quercetin, rutin, cyanidin, cyanidin-3-o-glucoside, delphinidin, delphinidin-3-o-glucoside have also been isolated from its extract.96,97 The extract has already been used for synthesis of Ag and AuNPs97,98 and shows potential for the synthesis of PdNPs as well and should be investigated. Similarly, in another study, fruit extract of Ficus carica has been used for the synthesis of silver and gold NPs and since the extract is found to contain flavonoids, proteins, sugars, and amino acids, it shows potential for biogenic synthesis of PdNPs and should also be investigated.99 Last but not the least, Cherries (Prunus avium L.) are also found to be rich in polyphenols and flavonoids and show great potential for the synthesis of PdNPs and should be investigated.100–102
Table 4 List of plants used for the synthesis of bimetallic NPs
Plant |
NPs |
Part used |
Size/shape of NPs |
Biomolecule involved |
Reference |
Euphorbia condylocarpa M. Bieb |
Pd/Fe3O4 |
Roots |
39 nm, shape not given |
Flavonoid and phenolic acids |
90 |
Theobroma cacao L. |
Pd–CuO |
Seeds |
40 nm, shape not given |
Phenolics |
88 |
Myrtus communis L. |
Pd/TiO2 |
Leaves |
17–25 nm, spherical |
Flavonoid/phenolics |
87 |
Euphorbia stracheyi Boiss |
Pd/Fe3O4 |
Roots |
10 nm, spherical |
Polyphenolics, flavonoid |
89 |
Cacumen platycladi |
Au–Pd |
Leaves |
7.4 nm, spherical |
Flavonoid and reducing sugars |
86 |
Withania coagulans |
Pd/RGO/Fe3O4 |
Leaves |
13 nm, spherical |
Flavonoids/other phenolics |
91 |
3 Conclusions
In order to avoid environmental contamination caused by the chemicals, high cost equipment and to save the energy used in chemical/physical synthetic methods, green synthesis of metallic NPs is of significant importance and has attracted remarkable attention of the scientific community. The process for the synthesis of metallic NPs, in particular PdNPs, using plant extract is easy, facile, low cost and environmental friendly. In such biogenic synthesis process, shape and size of the metallic NPs can be controlled by controlling the stoichiometry of metal, plant extract, pH and temperature. The naturally occurring structure property relationship of different phytomolecules can be exploited to synthesize new materials. Additionally, as a prospect, NPs can be produced on large scale by using crude plant extracts and its purified metabolites. Plants mediated production of PdNPs also shows significant advantages over microbial synthesis process. Literature survey shows a major body of published work on the green synthesis of silver and gold NPs in comparison to PdNPs and hence special attention is required to explore environmental friendly, economically viable and commercially feasible routes for the synthesis of PdNPs through natural reducing and stabilizing agents. Palladium is used as an important catalyst for various coupling reactions in research, in the chemical industry and its cheaper synthesis would affect cost of production for number of chemicals.
Acknowledgements
Z. H. gratefully acknowledges financial support from the Higher Education Commission (HEC) of Pakistan under NRPU project No. 20-3066/NRPU/R&D/HEC/13.
References
- S. Iravani, Green Chem., 2011, 13, 2638–2650 RSC.
- O. V. Kharissova, H. V. Dias, B. I. Kharisov, B. O. Perez and V. M. Perez, Trends Biotechnol., 2013, 31, 240–248 CrossRef CAS PubMed.
- P. Raveendran, J. Fu and S. L. Wallen, J. Am. Chem. Soc., 2003, 125, 13940–13941 CrossRef CAS PubMed.
- S. B. Kalidindi and B. R. Jagirdar, ChemSusChem, 2012, 5, 65–75 CrossRef CAS PubMed.
- R. S. Varma, Curr. Opin. Chem. Eng., 2012, 1, 123–128 CrossRef CAS.
- M. N. Tahir, P. Theato, P. Oberle, G. Melnyk, S. Faiss, U. Kolb, A. Janshoff, M. Stepputat and W. Tremel, Langmuir, 2006, 22, 5209–5212 CrossRef CAS PubMed.
- M. N. Tahir, L. Gorgishvili, J. Li, T. Gorelik, U. Kolb, L. Nasdala and W. Tremel, Solid State Sci., 2007, 9, 1105–1109 CrossRef CAS.
- M. N. Tahir, J. Herzberger, F. Natalio, O. Köhler, R. Branscheid, E. Mugnaioli, V. Ksenofontov, M. Penthofer, U. Kolb, H. Frey and W. Tremel, Nanoscale, 2016, 8, 9548–9555 RSC.
- M. N. Tahir, F. Natalio, M. A. Cambaz, M. Panthöfer, R. Branscheid, U. Kolb and W. Tremel, Nanoscale, 2013, 5, 9944–9949 RSC.
- S. Wooh, H. Huesmann, M. N. Tahir, M. Paven, K. Wichmann, D. Vollmer, W. Tremel, P. Papadopoulos and H. J. Butt, Adv. Mater., 2015, 27, 7338–7343 CrossRef CAS PubMed.
- K. N. Thakkar, S. S. Mhatre and R. Y. Parikh, Nanomedicine, 2010, 6, 257–262 CrossRef CAS PubMed.
- R. Rajan, K. Chandran, S. L. Harper, S.-I. Yun and P. T. Kalaichelvan, Ind. Crops Prod., 2015, 70, 356–373 CrossRef CAS.
- S. Ahmed, M. Ahmad, B. L. Swami and S. Ikram, J. Adv. Res., 2016, 7, 17–28 CrossRef CAS PubMed.
- C. Marambio-Jones and E. M. Hoek, J. Nanopart. Res., 2010, 12, 1531–1551 CrossRef.
- P. Mukherjee, A. Ahmad, D. Mandal, S. Senapati, S. R. Sainkar, M. I. Khan, R. Parishcha, P. Ajaykumar, M. Alam and R. Kumar, Nano Lett., 2001, 1, 515–519 CrossRef CAS.
- A. R. Shahverdi, S. Minaeian, H. R. Shahverdi, H. Jamalifar and A.-A. Nohi, Process Biochem., 2007, 42, 919–923 CrossRef.
- S. S. Shankar, A. Rai, A. Ahmad and M. Sastry, J. Colloid Interface Sci., 2004, 275, 496–502 CrossRef CAS PubMed.
- J. Y. Song and B. S. Kim, Bioprocess Biosyst. Eng., 2009, 32, 79–84 CrossRef CAS PubMed.
- K. B. Narayanan and N. Sakthivel, Adv. Colloid Interface Sci., 2010, 156, 1–13 CrossRef CAS PubMed.
- D. Mandal, M. E. Bolander, D. Mukhopadhyay, G. Sarkar and P. Mukherjee, Appl. Microbiol. Biotechnol., 2006, 69, 485–492 CrossRef CAS PubMed.
- D. MubarakAli, N. Thajuddin, K. Jeganathan and M. Gunasekaran, Colloids Surf., B, 2011, 85, 360–365 CrossRef CAS PubMed.
- J. Y. Song, E. Y. Kwon and B. S. Kim, Bioprocess Biosyst. Eng., 2010, 33, 159–164 CrossRef CAS PubMed.
- N. Dimitratos, F. Porta and L. Prati, Appl. Catal., A, 2005, 291, 210–214 CrossRef CAS.
- M. Sankar, N. Dimitratos, D. W. Knight, A. F. Carley, R. Tiruvalam, C. J. Kiely, D. Thomas and G. J. Hutchings, ChemSusChem, 2009, 2, 1145–1151 CrossRef CAS PubMed.
- M. Beller, H. Fischer, K. Kühlein, C.-P. Reisinger and W. Herrmann, J. Organomet. Chem., 1996, 520, 257–259 CrossRef CAS.
- S. Cheong, J. D. Watt and R. D. Tilley, Nanoscale, 2010, 2, 2045–2053 RSC.
- Y. Niu, L. K. Yeung and R. M. Crooks, J. Am. Chem. Soc., 2001, 123, 6840–6846 CrossRef CAS.
- P. K. Mandali and D. K. Chand, Catal. Commun., 2013, 31, 16–20 CrossRef CAS.
- K. Yu, W. Sommer, M. Weck and C. W. Jones, J. Catal., 2004, 226, 101–110 CrossRef CAS.
- L. S. Søbjerg, D. Gauthier, A. T. Lindhardt, M. Bunge, K. Finster, R. L. Meyer and T. Skrydstrup, Green Chem., 2009, 11, 2041 RSC.
- S. U. Son, Y. Jang, J. Park, H. B. Na, H. M. Park, H. J. Yun, J. Lee and T. Hyeon, J. Am. Chem. Soc., 2004, 126, 5026–5027 CrossRef CAS PubMed.
- V. Calo, A. Nacci, A. Monopoli and F. Montingelli, J. Org. Chem., 2005, 70, 6040–6044 CrossRef CAS PubMed.
- V. L. Nguyen, D. C. Nguyen, H. Hirata, M. Ohtaki, T. Hayakawa and M. Nogami, Adv. Nat. Sci.: Nanosci. Nanotechnol., 2010, 1, 035012 Search PubMed.
- F. Bonet, V. Delmas, S. Grugeon, R. Herrera Urbina, P. Y. Silvert and K. Tekaia-Elhsissen, Nanostruct. Mater., 1999, 11, 1277–1284 CrossRef CAS.
- W. Zhang, X. Qiao and J. Chen, Mater. Sci. Eng., B, 2007, 142, 1–15 CrossRef CAS.
- J. R. Peralta-Videa, L. Zhao, M. L. Lopez-Moreno, G. de la Rosa, J. Hong and J. L. Gardea-Torresdey, J. Hazard. Mater., 2011, 186, 1–15 CrossRef CAS PubMed.
- M. D. Yates, R. D. Cusick and B. E. Logan, ACS Sustainable Chem. Eng., 2013, 1, 1165–1171 CrossRef CAS.
- K. Deplanche, I. Caldelari, I. P. Mikheenko, F. Sargent and L. E. Macaskie, Microbiology, 2010, 156, 2630–2640 CrossRef CAS PubMed.
- J. R. Lloyd, P. Yong and L. E. Macaskie, Appl. Environ. Microbiol., 1998, 64, 4607–4609 CAS.
- W. D. Windt, P. Aelterman and W. Verstraete, Environ. Microbiol., 2005, 7, 314–325 CrossRef PubMed.
- T. Hennebel, S. Van Nevel, S. Verschuere, S. De Corte, B. De Gusseme, C. Cuvelier, J. P. Fitts, D. van der Lelie, N. Boon and W. Verstraete, Appl. Microbiol. Biotechnol., 2011, 91, 1435–1445 CrossRef CAS PubMed.
- M. N. Nadagouda, V. Polshettiwar and R. S. Varma, J. Mater. Chem., 2009, 19, 2026–2031 RSC.
- B. V. Tangahu, S. R. Sheikh Abdullah, H. Basri, M. Idris, N. Anuar and M. Mukhlisin, Int. J. Chem. Eng., 2011, 2011, 1–31 CrossRef.
- H. Ali, E. Khan and M. A. Sajad, Chemosphere, 2013, 91, 869–881 CrossRef CAS PubMed.
- A. K. Gupta, S. K. Verma, K. Khan and R. K. Verma, Environ. Sci. Technol., 2013, 47, 10115–10116 CAS.
- J. L. Gardea-Torresdey, E. Gomez, J. R. Peralta-Videa, J. G. Parsons, H. Troiani and M. Jose-Yacaman, Langmuir, 2003, 19, 1357–1361 CrossRef CAS.
- A. T. Harris and R. Bali, J. Nanopart. Res., 2008, 10, 691–695 CrossRef CAS.
- R. Haverkamp and A. Marshall, J. Nanopart. Res., 2009, 11, 1453–1463 CrossRef CAS.
- M. Khan, M. Khan, S. F. Adil, M. N. Tahir, W. Tremel, H. Z. Alkhathlan, A. Al-Warthan and M. R. H. Siddiqui, Int. J. Nanomed., 2013, 8, 1507–1516 Search PubMed.
- M. Khan, M. Khan, M. Kuniyil, S. F. Adil, A. Al-Warthan, H. Z. Alkhathlan, W. Tremel, M. N. Tahir and M. R. H. Siddiqui, Dalton Trans., 2014, 43, 9026–9031 RSC.
- A. H. Al-Marri, M. Khan, M. Khan, S. F. Adil, A. Al-Warthan, H. Z. Alkhathlan, W. Tremel, J. P. Labis, M. R. H. Siddiqui and M. N. Tahir, Int. J. Mol. Sci., 2015, 16, 1131–1142 CrossRef CAS PubMed.
- D. Sheny, D. Philip and J. Mathew, Spectrochim. Acta, Part A, 2012, 91, 35–38 CrossRef CAS PubMed.
- X. Yang, Q. Li, H. Wang, J. Huang, L. Lin, W. Wang, D. Sun, Y. Su, J. B. Opiyo, L. Hong, Y. Wang, N. He and L. Jia, J. Nanopart. Res., 2009, 12, 1589–1598 CrossRef.
- R. Kumar Petla, S. Vivekanandhan, M. Misra, A. Kumar Mohanty and N. Satyanarayana, J. Biomater. Nanobiotechnol., 2012, 03, 14–19 CrossRef.
- N. Edayadulla, N. Basavegowda and Y. R. Lee, J. Ind. Eng. Chem., 2015, 21, 1365–1372 CrossRef CAS.
- A. Kalaiselvi, S. M. Roopan, G. Madhumitha, C. Ramalingam and G. Elango, Spectrochim. Acta, Part A, 2015, 135, 116–119 CrossRef CAS PubMed.
- P. Dauthal and M. Mukhopadhyay, Ind. Eng. Chem. Res., 2013, 52, 18131–18139 CrossRef CAS.
- V. Vilas, D. Philip and J. Mathew, Mater. Chem. Phys., 2016, 170, 1–11 CrossRef CAS.
- M. Nasrollahzadeh and S. M. Sajadi, J. Colloid Interface Sci., 2016, 462, 243–251 CrossRef CAS PubMed.
- M. Nasrollahzadeh, S. M. Sajadi and M. Maham, J. Mol. Catal. A: Chem., 2015, 396, 297–303 CrossRef CAS.
- K. Mallikarjuna, N. John Sushma, B. V. Subba Reddy, G. Narasimha and B. Deva Prasad Raju, Int. J. Chem. Anal. Sci., 2013, 4, 14–18 CrossRef CAS.
- A. Kanchana, S. Devarajan and S. R. Ayyappan, Nano-Micro Lett., 2010, 2, 169–176 CrossRef CAS.
- B. Kumar, K. Smita, L. Cumbal and A. Debut, J. Saudi Chem. Soc., 2015, 19, 574–580 CrossRef.
- M. Nasrollahzadeh, S. M. Sajadi, A. Rostami-Vartooni, M. Alizadeh and M. Bagherzadeh, J. Colloid Interface Sci., 2016, 466, 360–368 CrossRef CAS PubMed.
- R. Molaie, K. Farhadi, M. Forough and R. Emamali, Proc. 4th Int. Conf. Nanostr. (ICNS4), 2012, pp. 12–14 Search PubMed.
- K. M. Kumar, B. K. Mandal, K. S. Kumar, P. S. Reddy and B. Sreedhar, Spectrochim. Acta, Part A, 2013, 102, 128–133 CrossRef PubMed.
- B. Baruwati and R. S. Varma, ChemSusChem, 2009, 2, 1041–1044 CrossRef CAS PubMed.
- L. Jia, Q. Zhang, Q. Li and H. Song, Nanotechnology, 2009, 20, 385601 CrossRef PubMed.
- S. M. Roopan, A. Bharathi, R. Kumar, V. G. Khanna and A. Prabhakarn, Colloids Surf., B, 2012, 92, 209–212 CrossRef CAS PubMed.
- A. Bankar, B. Joshi, A. R. Kumar and S. Zinjarde, Mater. Lett., 2010, 64, 1951–1953 CrossRef CAS.
- S. U. Ganaie, T. Abbasi and S. A. Abbasi, Part. Sci. Technol., 2015, 1–8, DOI:10.1080/02726351.2015.1058874.
- M. N. Nadagouda and R. S. Varma, Green Chem., 2008, 10, 859–862 RSC.
- R. W. Raut, A. S. M. Haroon, Y. S. Malghe, B. T. Nikam and S. B. Kashid, Adv. Mater. Lett., 2013, 4, 650–654 CrossRef CAS.
- M. Sathishkumar, K. Sneha, I. S. Kwak, J. Mao, S. Tripathy and Y.-S. Yun, J. Hazard. Mater., 2009, 171, 400–404 CrossRef CAS PubMed.
- D. Baruah, R. N. Das, S. Hazarika and D. Konwar, Catal. Commun., 2015, 72, 73–80 CrossRef CAS.
- A. J. Kora and L. Rastogi, Arabian J. Chem., 2015 DOI:10.1016/j.arabjc.2015.06.024.
- A. J. Kora and L. Rastogi, Arabian Ind. Crops Prod., 2016, 81, 1–10 CrossRef CAS.
- M. Nasrollahzadeh, M. Maham and M. M. Tohidi, J. Mol. Catal. A: Chem., 2014, 391, 83–87 CrossRef CAS.
- K. Farhadi, A. Pourhossein, M. Forough, R. Molaei, A. Abdi and A. Siyami, J. Chin. Chem. Soc., 2013, 60, 1144–1149 CrossRef CAS.
- J. Kou and R. S. Varma, RSC Adv., 2012, 2, 10283–10290 RSC.
- B. S. Naveen Prasad, T. V. N. Padmesh, V. Ganesh Kumar and K. Govindaraju, Int. J. Pharma Bio Sci., 2014, 5, 553–558 Search PubMed.
- M. Sathishkumar, K. Sneha and Y. Yun, Int. J. Mater. Sci., 2009, 4, 11–17 Search PubMed.
- B. S. N. Prasad and T.N. Padmesh, Int. J. Sci. Eng. Res., 2014, 5(3), 229–231 Search PubMed.
- R. Lakshmipathy, B. P. Reddy, N. Sarada, K. Chidambaram and S. K. Pasha, Appl. Nanosci., 2014, 5, 223–228 CrossRef.
- F. Luo, D. Yang, Z. Chen, M. Megharaj and R. Naidu, J. Hazard. Mater., 2016, 303, 145–153 CrossRef CAS PubMed.
- G. Zhan, J. Huang, M. Du, I. Abdul-Rauf, Y. Ma and Q. Li, Mater. Lett., 2011, 65, 2989–2991 CrossRef CAS.
- M. Nasrollahzadeh and S. Mohammad Sajadi, J. Colloid Interface Sci., 2015, 465, 121–127 CrossRef PubMed.
- M. Nasrollahzadeh, S. M. Sajadi, A. Rostami-Vartooni and M. Bagherzadeh, J. Colloid Interface Sci., 2015, 448, 106–113 CrossRef CAS PubMed.
- M. Nasrollahzadeh and S. M. Sajadi, J. Colloid Interface Sci., 2016, 464, 147–152 CrossRef CAS PubMed.
- M. Nasrollahzadeh, S. Mohammad Sajadi, A. Rostami-Vartooni and M. Khalaj, J. Mol. Catal. A: Chem., 2015, 396, 31–39 CrossRef CAS.
- M. Atarod, M. Nasrollahzadeh and S. M. Sajadi, J. Colloid Interface Sci., 2016, 465, 249–258 CrossRef CAS PubMed.
- S. Umoren, I. Obot and Z. Gasem, J. Mater. Environ. Sci., 2014, 5, 907–914 Search PubMed.
- N. Kumar, P. Bhandari, B. Singh, A. P. Gupta and V. K. Kaul, J. Sep. Sci., 2008, 31, 262–267 CrossRef CAS PubMed.
- S. M. Ghoreishi, M. Behpour and M. Khayatkashani, Phys. E, 2011, 44, 97–104 CrossRef CAS.
- S. Sreekumar, H. Sithul, P. Muraleedharan, J. M. Azeez and S. Sreeharshan, BioMed Res. Int., 2014, 2014, 686921 Search PubMed.
- C. V. S. Prakash and I. Prakash, Int. J. Res. Chem. Environ., 2011, 1, 1–18 Search PubMed.
- S. S. Dash and B. G. Bag, Appl. Nanosci., 2012, 4, 55–59 CrossRef.
- M. N. Nadagouda, N. Iyanna, J. Lalley, C. Han, D. D. Dionysiou and R. S. Varma, ACS Sustainable Chem. Eng., 2014, 2, 1717–1723 CrossRef CAS.
- P. P. Singh and C. Bhakat, International Journals of Scientific Research Publications, 2012, 2, 1–4 Search PubMed.
- D. Prvulović, M. Popović, Đ. Malencic, M. Ljubojvic and V. Ognjanov, Int. J. Agr. Sci. Res., 2011, 43, 2 Search PubMed.
- B. Gonçalves, A.-K. Landbo, D. Knudsen, A. P. Silva, J. Moutinho-Pereira, E. Rosa and A. S. Meyer, J. Agric. Food Chem., 2004, 52, 523–530 CrossRef PubMed.
- L. Gao and G. Mazza, J. Agric. Food Chem., 1995, 43, 343–346 CrossRef CAS.
|
This journal is © The Royal Society of Chemistry 2016 |
Click here to see how this site uses Cookies. View our privacy policy here.