DOI:
10.1039/C6RA11735J
(Paper)
RSC Adv., 2016,
6, 58485-58492
Design of controlled multi-probe coupled assay via bioinspired signal amplification approach for mercury detection†
Received
5th May 2016
, Accepted 3rd June 2016
First published on 7th June 2016
Abstract
We have designed and synthesized off–on fluorometric probes, 7-(but-3-yn-1-yloxy)-8-(difluoromethyl)-2H-chromen-2-one (AYF) and 7-((4-(tert-butyldiphenylsilyloxy)benzyl)oxy)-8-(difluoromethyl)coumarin (DPF1), by incorporating the concept of biological signal transduction strategy into their molecular design and present their proof-of-concept demonstration for mercury (Hg2+) detection. AYF undergoes a cascade of self-immolative reactions in the presence of Hg2+ and unmasks fluorogenic coumarin 1 and releases two latent fluoride ions. These fluorides initiate the removal of silyl protecting trigger group on DPF1 which directs another cascade of self-immolative reactions. The fluoride ions (amplifiers) are continuously activating the cascade reaction, and, as a result, coumarin 1 progressively accumulates in the reaction medium and the whole process accelerates an amplified signal for Hg2+. For detection of Hg2+, the limit of detection of the two-probe coupling assay (AYF and DPF1) is 0.51 ppb which is more than 2000 times lower than that of AYF alone. Besides, 3-(benzothiazol-2-yl)-4-carbonitrile-7-((4-(tert-butyldiphenylsilyloxy)benzyl)oxy)coumarin (DCC), a long-wavelength probe, was coupled with AYF and DPF1 in order to shift the emission to a higher wavelength region. Negative control studies revealed that the probes undergo controlled and organized sensing pathways as we designed.
1. Introduction
The development of novel sensing approaches and methods in order to improve sensitivity and selectivity of analytical methods is an active area of research in analytical chemistry.1 A sensing method seeks to change unknown information (identity, quality, reactivity, etc.) in a chemical or biological sample into measurable signals. The signals can be obtained from various transduction techniques, where the unknown information from the sample is then transformed into optical,2,3 electrical,4,5 or mechanical6 readouts. The currently known sensitive and selective techniques such as enzyme-linked immunosorbent assay (ELISA),7 polymerase chain reaction (PCR),8 and bio-barcode assays9 usually rely on antibodies, enzymes or biomolecules10 to achieve high sensitivity and selectivity. However, in most cases these reagents lack thermal stability and are not stable for prolonged periods; as a result they encounter practical difficulties in performing real-application analysis at ambient temperature. In order to circumvent these limitations, recently several research groups have reported the design of auto-inductive molecular probes by incorporating the signal amplification concept of biomolecules to achieve high sensitivity and selectivity.11–16 Biological systems recognize and respond to external signals or stimulants through signal transduction processes, where the original signal is amplified into other chemical information through well-organized catalytic processes.17 Generally, the auto-inductive molecular probes are equipped with unique triggering groups which selectively interact with the analyte of interest via a designated chemical reactions pathway. The analyte induces the designated chemical reactions which leads to a cascade of self-immolative reactions and unmasks the reporter molecules (chromogenic or fluorogenic) and spontaneously liberates the signal transduction molecules. These signal transduction molecules continuously induce the auto-inducible molecular probes to undergo self-immolative signal-revealing reactions which accumulate reporter molecules, and this cyclic process facilitates amplification (Scheme 1).
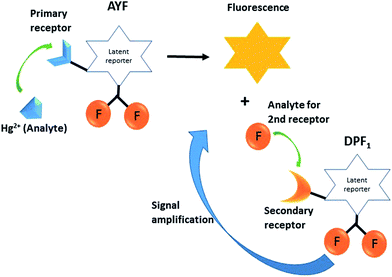 |
| Scheme 1 A general scheme for signal amplification strategy. | |
Here, we sought to design auto-inductive fluorescent probes by incorporating the biological signal transduction concept and demonstrate their proof-of-concept for mercury ion (Hg2+) detection. Hg2+ is one of the most toxic and highly poisonous metal ions and a widespread pollutant of natural and anthropogenic sources. Organic forms of mercury, such as methylmercury species, are much more toxic than inorganic mercury species.18 Notably, methylmercury species readily cross the blood–brain barrier due to their lipid solubility and accumulate in the brain which can cause damage to the central nervous system and other organs as well.19,20 Thus, the active monitoring of Hg2+ in our environment is needed to ensure proper physiological function and food safety,21 and the development of novel approaches for the sensitive detection of Hg2+ is extremely important.22,23
Designing latent self-immolative ratiometric chemical probes for sensor applications is of ongoing interest in our research group.24–26 Recently, we have successfully designed an auto-inducible off–on fluorogenic ratiometric probe for ultrasensitive fluoride detection.25 We are interested in expanding the similar signal-revealing mechanism to design a new bioinspired sensing approach for Hg2+ detection using the auto-inductive off–on fluorogenic probes AYF and DPF1 (Scheme 2). The probe AYF is composed of a 3-butynyl group attached via ether linkage to the latent reporter coumarin which carries two fluorides, and these fluorides act as signal amplifiers. The Hg2+ facilitates the removal of the 3-butynyl group from AYF via oxymercuration reaction which results in a cascade of self-immolative reactions through quinone-methide type of rearrangement to generate fluorogenic reporter 8-formyl-7-hydroxycoumarin (coumarin 1),27,28 with concomitant release of two fluorides.29 Notably, Koide et al. successfully demonstrated the Hg2+-promoted cleavage of fluorescence-masking 3-butyne or vinyl groups in low ppb ranges under mild condition.27,28 The latent generated fluorides then induce the removal of silyl protecting trigger group on DPF1 which results in another cascade of self-immolative reactions in a similar way as AYF and eject coumarin 1 and two additional fluorides. The released fluorides are highly capable of continuously inducing the self-immolative reactions of unreacted DPF1, and this cyclic process leads to the accumulation of fluorogenic coumarin 1 which results in exponential signal amplification. The main objective of this work is to design a controlled multi-probe coupling assay incorporating the concept of biological signal transduction for Hg2+ detection and demonstrate its proof-of-concept for Hg2+. To our knowledge, this is the first report which incorporates the biological signal amplification concept for Hg2+ detection.
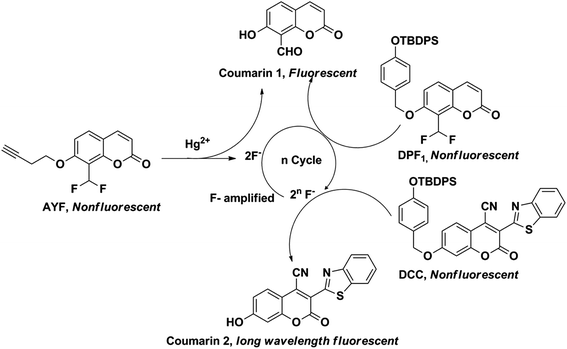 |
| Scheme 2 Pathway by which AYF detects Hg2+ and releases two equivalents of fluorides to propagate the auto-inductive signal amplification process. | |
2. Experimental
2.1. Chemicals and instrumentation
The chemicals were purchased from Acros Organics, Sigma-Aldrich, Showa Chemical Industry Co., or TCI America and were used without further purification. All reactions requiring anhydrous conditions were performed in oven-dried glassware under an Ar or N2 atmosphere. Chemicals and solvents were either puriss p.a. or purified by standard techniques. Analytical thin-layer chromatography was performed using glass plate-mounted silica gel 60F254 (Merck) with a thickness of 0.2 mm. Flash column chromatography was performed using Silicycle silica gel 60. The synthesized compounds were characterized by using 1H NMR (Bruker Advance 300 MHz) and IR spectroscopy (HORIBA 720 infrared spectrophotometer). Mass spectra were recorded using a Finnigan TSQ 700 triple quadrupole mass spectrometer equipped with an electrospray ionization (ESI) ion source. Photoluminescence spectra were measured with a HORIBA FluoroMax-4 spectrometer. 0.1 M citric acid BIS-TRIS propane buffer (CBTP) (pH 7.1 and 7.6) was prepared with CBTP in water.
2.2. Synthesis of 7-(but-3-yn-1-yloxy)-2-oxo-2H-chromene-8-carbaldehyde (2)
A mixture of 7-hydroxy-8-aldehydecoumarin (1, 0.05 g, 0.263 mmol) and potassium carbonate (K2CO3, 0.109 g, 0.788 mmol) was dissolved in 1.314 mL anhydrous N,N-dimethylformamide (DMF) with subsequent addition of 3-butynyl p-toluenesulfonate (0.175 mL, 0.788 mmol) to the mixture. The whole mixture was stirred under a stream of argon (Ar) at 84 °C for 24 h and the reaction mixture was extracted with dichloromethane (DCM)/water. The organic layer was dried over anhydrous magnesium sulfate (MgSO4). The solvent was removed and the residue was purified by column chromatography on silica gel (eluent: ethyl acetate/n-hexane = 1
:
1) yielding the title compound 2 as a white solid (78%, 0.0492 g). 1H NMR (CDCl3, 300 MHz, ppm): δ = 10.63 (s, 1H), 7.61 (t, J = 9.3, 2H), 6.91 (d, J = 8.7, 1H), 6.32 (d, J = 9.6, 1H), 4.26 (t, J = 6.9, 2H), 2.75 (m, 2H), 2.04 (t, J = 2.7, 1H). FTIR (cm−1): 3417.24, 3243.68, 2358.51, 1710.55, 1681.62, 1598.70, 1560.13, 1486.85, 1394.28, 1295.93, 1241.93, 1182.15, 1118.51, 1079.94, 831.17, 767.53. ESI-MS: m/z = 265 [M + H+]. Melting point: 166–168 °C.
2.3. Preparation of 7-(but-3-yn-1-yloxy)-8-(difluoromethyl)-2H-chromen-2-one (AYF)
7-(But-3-yn-1-yloxy)-2-oxo-2H-chromene-8-carbaldehyde (2, 0.3671 g, 1.53 mmol) was dissolved in 7.65 mL anhydrous DCM, and subsequently diethylaminosulfur trifluoride (DAST) (0.56 mL, 4.58 mmol) was added and stirred for 24 h under argon atmosphere at room temperature. Afterwards, the reaction mixture was extracted with DCM/water. The organic layer was dried over MgSO4. The solvent was removed and the residue was purified by flash chromatography on silica gel (ethyl acetate/n-hexane = 1
:
3) to obtain the title compound AYF as a light yellow solid (62%, 0.2496 g). 1H NMR (CDCl3, 300 MHz, ppm): δ = 7.62 (d, J = 9.3, 1H), 7.51 (d, J = 8.7, 1H), 7.26 (t, J = 53.1, 1H), 6.88 (d, 8.7, 1H), 6.30 (d, J = 9.6, 1H), 4.23 (t, J = 7.2, 2H), 2.74 (m, J = 11.4, 2H), 2.05 (t, J = 2.4, 1H). FTIR (cm−1): 3303.46, 3075.9, 2958.52, 2900.41, 1735.62, 1608.34, 1498.49, 1390.42, 1319.07, 1249.65, 1191.79, 1118.51, 1074.16, 1006.66, 838.88, 779.10, 725.10, 636.39, 566.97, 466.69. ESI-MS: m/z = 264 [M + H+]. Melting point: 159–161 °C.
2.4. Assay conditions for the detection of Hg2+ using AYF
A stock solution of AYF was prepared in dimethyl sulfoxide (DMSO). In a typical assay, AYF (100 μM) and Hg2+ were incubated in DMSO/CBTP (pH = 7.6, 10 mM)/acetonitrile (ACN) (7
:
2
:
1, v/v/v) solution at the respective temperature and time. Various concentrations of standard Hg2+ ions or other metal ions (in the case of selectivity studies) were added, and the release of 7-hydroxy-8-formylcoumarin (coumarin 1) was monitored by recording fluorescence spectra at λex = 360 nm and λem = 460 nm. The assay consists of two steps: (1) incubation of AYF in the solution containing DMSO and CBTP for 3 h at 90 °C and (2) ACN was added and incubated in a water bath for 1 h at 60 °C.
2.5. Assay conditions for the detection of Hg2+ using two-probe coupling assay (AYF and DPF1)
The probe DPF1 (7-((4-(tert-butyldiphenylsilyloxy)benzyl)-oxy)-8-(difluoromethyl)coumarin) was synthesized according to our previous report.25 A stock solution of DPF1 was prepared in ACN. The assay consists of two steps: (1) incubation of AYF (200 μM) and Hg2+ in 100 μL of DMSO/CBTP (pH = 7.1, 10 mM) (9
:
1, v/v) at 90 °C for 3 h and (2) addition of DPF1 (50 μM) in ACN/pyridine (89
:
1, v/v) until the total volume of sample was 1 mL and incubation in a water bath for 3 h at 40 °C. Different concentrations of Hg2+ were added and the release of coumarin 1 was monitored.
2.6. Assay conditions for the detection of Hg2+ using AYF and DCC
A stock solution of DCC was prepared in ACN. The assay consists of two steps: (1) AYF (100 μM) and Hg2+ were incubated in the solution containing DMSO and CBTP (pH = 7.6, 10 mM) for 3 h at 90 °C and (2) DCC (5 μM) in ACN was added and incubated in a water bath at 60 °C for 1 h. The release of coumarin 1 was monitored at λex = 360 nm and λem = 460 nm, while the release of 3-(2′-benzothiazolyl)-4-carbonitrile-7-hydroxycoumarin (coumarin 2) was monitored at λex = 500 nm and λem = 600 nm.
2.7. Assay conditions for the detection of Hg2+ using three-probe coupling assay (AYF, DPF1 and DCC)
The probe DCC (3-(benzothiazol-2-yl)-4-carbonitrile-7-((4-(tert-butyldiphenylsilyloxy)benzyl)oxy)coumarin) was prepared in accordance with our reported procedure.25 Three-probe assay testing consists of two steps: (1) AYF (200 μM) and Hg2+ were incubated in 100 μL solution of DMSO/CBTP (pH = 7.1, 10 mM) (9
:
1, v/v) for 3 h at 90 °C and (2) DPF1 (50 μM) and DCC (20 μM) in ACN/pyridine (89
:
1, v/v) were added and the whole solution (1 mL) was incubated in a water bath for 3 h at 40 °C. Various concentrations of standard Hg2+ ions were tested and the release of coumarin 2 was monitored.
3. Results and discussion
The synthesis of AYF is outlined in Scheme 3. A two-step synthesis from coumarin 1 gave the desired AYF. Coupling coumarin 1 with but-3-yn-1-yl-4-methylbenzenesulfonate under basic condition gave the alkylated ether 2 in 78% yield. Treatment of aldehyde 2 with DAST furnished the geminal di-fluorides and the resulting compound was named as AYF. The overall yield of the two steps was 48%. The chemical structures of the synthetic intermediates and the final products were determined by NMR and IR spectroscopy and mass spectrometry.
 |
| Scheme 3 Synthesis of AYF. | |
3.1. Detection of mercury with AYF
Under the same experimental conditions, the pristine analogue coumarin 1 is able to produce a highly intensive fluorescence signal (inset Fig. 1A), while AYF is unable to produce any signal. As expected, the fluorophore (coumarin 1) of AYF is completely masked by the specially designed trigger group. In the presence of Hg2+, AYF produced an obvious fluorescence signal corresponding to the emission spectrum of coumarin 1 (Fig. 1A). As explained in an earlier section, AYF undergoes oxymercuration in the presence of Hg2+ which unmasks and releases coumarin 1 which produces a fluorescence signal (Scheme 2). Furthermore, the sensing ability of AYF in the presence of different concentrations of Hg2+ was determined by recording its fluorescence spectra (λex = 360 nm and λem = 460 nm) by following the assay conditions given in Section 2.4. The fluorescence intensity increases linearly as the concentration of Hg2+ increases. A plot between fluorescence intensity and concentration of Hg2+ exhibits a linear relationship in a concentration range of 2 ppm–10 ppm (Fig. 1B). The limit of detection (LOD) of AYF to detect Hg2+ was calculated to be 1.2 ppm. The LOD was calculated using the formula LOD = 3sb/S, where sb is the standard deviation of ten blank measurements and S is the sensitivity. The selectivity of AYF towards detection of Hg2+ in the presence of other metal ions was examined (Fig. 1C). AYF delivered well-defined fluorescence signal for 10 ppm Hg2+, whereas no significant fluorescence responses were observed for the other metal ions (Pd2+, Cu2+, Mg2+, Sr2+, Pb2+, Cd2+, Co2+ and Ni2+). Therefore, the probe AYF is suitable for Hg2+ detection in the presence of other metal ions.
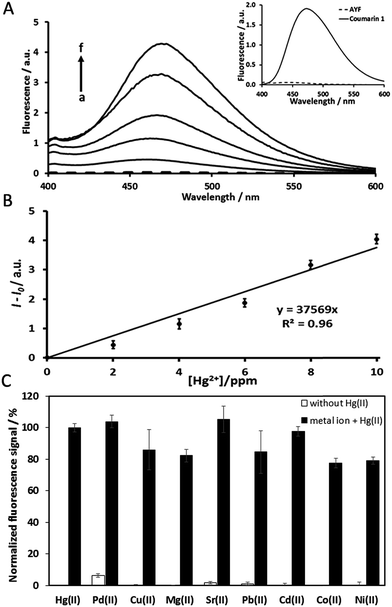 |
| Fig. 1 (A) Fluorescence enhancement (intensity difference I − I0/a.u.) of AYF (100 μM) with Hg2+ (a = 0, b = 2, c = 4, d = 6, e = 8, f = 10 ppm) in 20% CBTP buffer (pH = 7.6, 10 mM) containing 70% DMSO and 10% can (inset: fluorescence emission spectra of AYF and pristine coumarin 1). I = intensity of the fluorescence signal at a particular concentration of Hg2+, I0 = intensity of blank sample. (B) Linear calibration plot: I − I0/a.u. vs. [Hg2+]/ppm. (C) Fluorescence emission change of AYF (100 μM) in the presence of other metal ions. The white bars represent the emission intensity in the presence of 10 ppm of the metal ion of interest. The black bars represent the change of the emission intensity in the presence of 10 ppm Hg2+ and 10 ppm of the metal ion of interest. Excitation was at 360 nm and the emission intensities were recorded at 460 nm. Error bars represent the standard deviation of three individual measurements. | |
3.2. Detection of mercury with AYF coupled with autocatalytic signal amplification (AYF + DPF1)
In order to improve the sensitivity of AYF, we have incorporated a signal amplification strategy by using a two-probe coupling assay: AYF and DPF1. The sensing ability of this coupling assay was determined by recording fluorescence spectra (λex = 360 nm, λem = 445 nm) at different concentrations of Hg2+. The assay conditions were followed as given in Section 2.5. As shown in Fig. 2, the fluorescence intensity increases linearly as the concentration of Hg2+ increases and the plot between fluorescence intensity and [Hg2+] exhibits a linear relationship in a concentration range of 1–5 ppb. The LOD for Hg2+ using AYF coupled with DPF1 is calculated to be 0.51 ppb which is more than 2000 times lower than that obtained for AYF alone. This must be due to the signal amplification through the cascade of self-immolative reactions which were induced by the latent generated fluorides as explained in Scheme 2. Thus, the fluorescence determination studies clearly revealed that the designed probes followed the proposed mechanistic pathway which rendered a low LOD as expected. It is worth mentioning that a previously reported fluorescein probe which was designed based on the reactivity of Hg2+ with alkynes was able to reach a low detection range of 8 ppb.28 Interestingly, our two-probe coupling assay (AYF and DPF1) incorporating a signal amplification strategy is able to reach a LOD of 0.51 ppb which is roughly 14 times lower than that of the previously reported fluorescein-based probe for Hg2+.
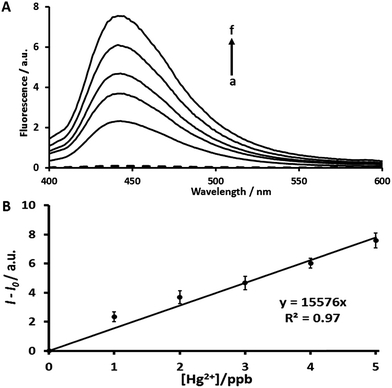 |
| Fig. 2 (A) Fluorescence enhancement (intensity difference/a.u.) of two-probe coupling assay (AYF and DPF1) with different concentrations of Hg2+ (a = 0, b = 1, c = 2, d = 3, e = 4, f = 5 ppb). (B) Linear calibration plot: I − I0/a.u. vs. [Hg2+]/ppb. Error bars represent the standard deviation of three individual measurements. | |
In order to determine whether Hg2+ ions cleave DPF1, we have carried out negative control experiments with DPF1 alone in the presence and absence of Hg2+ (Fig. S7†). As seen from the figure, DPF1 alone is unable to produce a significantly different fluorescence signal even in the presence of Hg2+ indicating that Hg2+ had no reaction with DPF1. Hg2+ ions selectively cleave AYF and produce two fluoride ions which react with the DPF1 and produce signal amplification. Therefore, the experimental results reveal that the designed probes follow the controlled sensing pathway as described in the schematic mechanism.
3.3. Mercury detection with long-wavelength fluorogenic probe
The use of a long-wavelength fluorogenic probe is an attractive alternative to conventional UV-visible fluorophores because fluorescence occurs in the long-wavelength region of the electromagnetic spectrum and can avoid auto-fluorescence30 and minimize absorption or emission associated with other compounds that might be present in the sample. DCC is a fluorescent probe with maximum emission in the 595 nm region.31 Luminescence measurements in this region increase spectral selectivity because the interference of potential auto-fluorescence signals can be reduced.32 Using two small-molecule systems, AYF and DCC, we have examined the detection of Hg2+ in the long-wavelength region. The two-probe coupling assay (AYF and DCC) exhibits highly enhanced fluorescence signal for each addition of Hg2+ (Fig. 3A). The assay conditions for the detection of Hg2+ using AYF and DCC coupling assay are given in Section 2.6. The fluorescence intensity linearly increases as the concentration of Hg2+ increases in a range from 1 to 5 ppm (Fig. 3B). As explained in Scheme 2, the two geminal fluorides ejected by AYF induce self-immolative reactions of DCC to release long-wavelength reporter coumarin 2. Along with the advantage of long-wavelength detection, this assay platform has also shown higher sensitivity than the assay platform using only AYF. Therefore, the AYF and DCC coupling assay will be a promising approach for the selective and sensitive detection of Hg2+ in biological samples. In order to determine whether Hg2+ ions cleave DCC, negative control experiments with DCC alone in the presence and absence of Hg2+ were carried out (Fig. S8†). As expected, DCC alone in the presence of Hg2+ is unable to elicit a fluorescence signal indicating that Hg2+ had no reaction with DCC (Fig. S8†). Thus, DCC follows the controlled sensing pathway as described in the schematic mechanism.
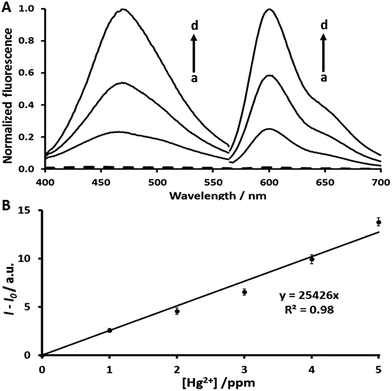 |
| Fig. 3 (A) Fluorescence emission changes (λex = 360 and 500 nm), consisting of AYF (100 μM) upon incubation with different concentrations of Hg2+ (a = 0, b = 6, c = 8, d = 10 ppm) in 20% CBTP buffer (pH = 7.6, 10 mM) and 70% DMSO for 3 h at 90 °C, and 10% ACN solution of DCC (5 μM) was added for 1 h at 60 °C. (B) Linear calibration plot for two-probe coupling assay (AYF + DCC). Error bars represent the standard deviation of three individual measurements. | |
After successfully developing the long-wavelength assay with AYF and DCC, then we investigated the use of DCC probe coupled with AYF as a mercury-sensitive probe and DPF1 as a signal amplification generator. The sensing ability of this three-probe coupling assay (AYF, DPF1 and DCC) was determined by recording fluorescence spectra (λex = 500 nm, λem = 595 nm) at different concentrations of Hg2+. The assay conditions were followed as given in Section 2.7. The intensity of fluorescence increases linearly as the concentration of Hg2+ increases and the plot between fluorescence intensity and [Hg2+] exhibits a linear relationship in a concentration range of 0.5–2.5 ppb (Fig. 4). The LOD for the three-probe assay is calculated to be 0.16 ppb (0.8 nM) which is lower than that for the two-probe assay (AYF and DPF1) and around 7000 times lower than that obtained for AYF alone. Also, the LOD of our three-probe coupled assay is well below the allowed level of Hg2+ in drinking water regulated by the US EPA. Previously reported fluorescent probes based on rhodamine–coumarin conjugate, rhodamine dye attached with a receptor composed of thiol and alkene and Nile blue displayed LODs of 40 nM, 27.5 nM and 1 ppb, respectively.33–35 In comparison with these previous probes, our three-probe coupling assay shows better sensitivity. Thus, the proof-of-concept of our bioinspired approach which uses Hg2+ as analyte and fluoride as signal transduction messenger as described in Schemes 1 and 2 is successfully validated. Although the sensor performance of our approach is not outstanding and requires the use of mixed solvents, it is interesting to develop novel approaches for Hg2+ detection by mimicking biological signal transduction pathways.
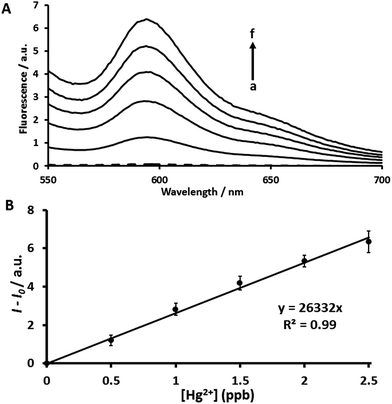 |
| Fig. 4 (A) Fluorescence enhancement (intensity difference/a.u.) of three-probe coupling assay (AYF, DPF1, and DCC) with different concentrations of Hg2+ (a = 0, b = 0.5, c = 1.0, d = 1.5, e = 2.0, f = 2.5 ppb). (B) Linear calibration plot: I − I0/a.u. vs. [Hg2+]/ppb. Error bars represent the standard deviation of three individual measurements. | |
3.4. The selectivity of the developed platform
Our previous study has shown that the DPF1 platform itself is highly specific for fluoride over other several common anions, including Cl−, Br−, I−, N3−, CH3COO−, HSO4−, NO3−, and H2PO4−.25 Here, we have investigated the selectivity of our developed platform in the presence of other metal ions. Several other metal ions, including Pd2+, Cu2+, Mg2+, Sr2+, Pb2+, Co2+, Cd2+, Ca2+, Ba2+, Fe2+, Mn2+, Ni2+, Zn2+, Ag+, and K+, were tested (10 equivalents of Hg2+) (Fig. 5). No significant spectral changes were observed in the presence of other metal ions in comparison with blank sample, and only Hg2+ elicited a strong fluorescence signal. These results reveal the specificity of the detection platform for Hg2+. Moreover, our results are similar to those for another fluorescence-masking alkyl probe to detect Hg2+ which indicate that the presence of other metal ions will not significantly increase fluorescence intensity.28
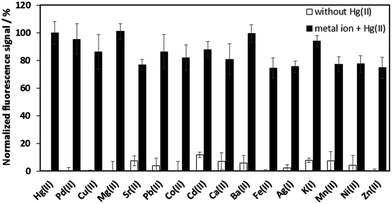 |
| Fig. 5 Fluorescence emission change of three-probe coupling assay platform, consisting of AYF (200 μM), DPF1 (50 μM), and DCC (20 μM), in the presence of other metal ions. The white bars represent the emission intensity in the presence of 1 ppm of the metal ion of interest (except for Mn2+, Ni2+ and Zn2+, 50 ppb). The black bars represent the change of emission intensity in the presence of 5 ppb Hg2+ and 1 ppm of the metal ion of interest (except for Mn2+, Ni2+ and Zn2+, 50 ppb). λex = 500 nm and emission intensities were recorded at 595 nm. Error bars represent the standard deviation of three individual measurements. | |
3.5. Real sample analysis
We have explored the real-time applicability of our three-probe coupled approach for tap water and bovine serum samples via the spiking method. The spiked Hg2+ concentrations are 10 and 40 ppb in tap water sample, while they are 100 and 150 ppb in bovine serum sample. The detailed experimental procedure for preparation of tap water and bovine serum samples is given in the ESI.† The amount of Hg2+ in final experimental medium is calculated by considering the dilution made in the first step of the assay, wherein dilution factors of 20 and 200 were considered for tap water and bovine serum, respectively. The added, calculated, found and recovery values are given in Table 1. The quantitative recoveries of spiked samples were satisfactory, which reveals the promising practical applicability and validates the proof-of-concept of our approach inspired from biological signal transduction pathways.
Table 1 Determination of mercury present in tap water and bovine serum samples using three-probe platform (AYF, DPF1, and DCC)
Sample |
Spiked/ppb |
Calculated amount of Hg2+ in final experimental medium/ppb |
Found/ppb |
Recovery/% |
RSDa |
RSD of three individual measurements. |
Tap water |
10 |
0.5 |
10.81 |
108.09 |
0.77 |
40 |
2 |
38.63 |
96.57 |
1.89 |
Bovine serum |
100 |
0.5 |
94.73 |
94.73 |
1.89 |
150 |
0.75 |
147.27 |
98.18 |
5.40 |
4. Conclusions
In summary, we have successfully demonstrated the proof-of-concept of the AYF, DPF1 and DCC coupled bioinspired fluorescence approach in the detection of Hg2+. The successful incorporation of the concept of a biological signal transduction strategy into the molecular design of probes was revealed by their fluorescence sensing ability towards Hg2+. The sensitivity of AYF to detect Hg2+ is greatly improved (about 7000 times) after it is coupled with DPF1 and DCC which validates the significance of the described approach towards enhancing the sensitivity of Hg2+ detection. Besides, the real-time analyses demonstrated for water and biological samples add additional validation to the proof-of-concept. Despite the average sensing performance of the approach over benchmark Hg2+ fluorescence sensors, biomimic approaches have the advantage of nature and accomplish complex and multiple goals. The described bioinspired controlled multi-probe sensing approach holds great promise for the detection of metal ions and small molecules.
Acknowledgements
This work was supported by the Ministry of Science and Technology, Taiwan (NSC 103-2811-M-027-002, 102-2113-M-027-002-MY3, and MOST 104-2622-M-027-001-CC3).
References
- D. A. Giljohann and C. A. Mirkin, Nature, 2009, 462, 461–464 CrossRef CAS PubMed.
- L. Zhu and E. V. Anslyn, J. Am. Chem. Soc., 2004, 126, 3676–3677 CrossRef CAS PubMed.
- J. F. Zhang, C. S. Lim, S. Bhuniya, B. R. Cho and J. S. Kim, Org. Lett., 2011, 13, 1190–1193 CrossRef CAS PubMed.
- J. Wang, G. Liu, B. Munge, L. Lin and Q. Zhu, Angew. Chem., Int. Ed., 2004, 43, 2158–2161 CrossRef CAS PubMed.
- C. Fan, K. W. Plaxco and A. J. Heeger, Proc. Natl. Acad. Sci. U. S. A., 2003, 100, 9134–9137 CrossRef CAS PubMed.
- J. Fritz, M. Baller, H. Lang, H. Rothuizen, P. Vettiger, E. Meyer, H.-J. Güntherodt, C. Gerber and J. Gimzewski, Science, 2000, 288, 316–318 CrossRef CAS PubMed.
- J. P. Gosling, Clin. Chem., 1990, 36, 1408–1427 CAS.
- N. Blow, Nat. Methods, 2007, 4, 869–875 CrossRef CAS.
- J.-M. Nam, C. S. Thaxton and C. A. Mirkin, Science, 2003, 301, 1884–1886 CrossRef CAS PubMed.
- P. Scrimin and L. J. Prins, Chem. Soc. Rev., 2011, 40, 4488–4505 RSC.
- N. Karton-Lifshin and D. Shabat, New J. Chem., 2012, 36, 386–393 RSC.
- M. S. Baker and S. T. Phillips, J. Am. Chem. Soc., 2011, 133, 5170–5173 CrossRef CAS PubMed.
- E. Sella, A. Lubelski, J. Klafter and D. Shabat, J. Am. Chem. Soc., 2010, 132, 3945–3952 CrossRef CAS PubMed.
- E. Sella, R. Weinstain, R. Erez, N. Z. Burns, P. S. Baran and D. Shabat, Chem. Commun., 2010, 46, 6575–6577 RSC.
- M. Avital-Shmilovici and D. Shabat, Bioorg. Med. Chem., 2010, 18, 3643–3647 CrossRef CAS PubMed.
- E. Sella and D. Shabat, J. Am. Chem. Soc., 2009, 131, 9934–9936 CrossRef CAS PubMed.
- S. Goggins, C. Naz, B. J. Marsh and C. G. Frost, Chem. Commun., 2015, 51, 561–564 RSC.
- J. Risher and R. DeWoskin, Public health services, Agency for toxic substances and disease registry, 1999 Search PubMed.
- T. W. Clarkson and L. Magos, CRC Crit. Rev. Toxicol., 2006, 36, 609–662 CrossRef CAS PubMed.
- F. Bakir, S. Damluji, L. Amin-Zaki, M. Murtadha, A. Khalidi, N. Al-Rawi, S. Tikriti, H. Dhahir, T. Clarkson and J. Smith, Science, 1973, 181, 230–241 CrossRef CAS PubMed.
- F. Di Natale, A. Lancia, A. Molino, M. Di Natale, D. Karatza and D. Musmarra, J. Hazard. Mater., 2006, 132, 220–225 CrossRef CAS PubMed.
- W. Lin, X. Cao, Y. Ding, L. Yuan and L. Long, Chem. Commun., 2010, 46, 3529–3531 RSC.
- W. Huang, X. Zhu, D. Wua, C. He, X. Hu and C. Duan, Dalton Trans., 2009, 10457–10465 RSC.
- J.-A. Gu, Y.-J. Lin, Y.-M. Chia, H.-Y. Lin and S.-T. Huang, Microchim. Acta, 2013, 180, 801–806 CrossRef CAS.
- J.-A. Gu, V. Mani and S.-T. Huang, Analyst, 2015, 140, 346–352 RSC.
- S.-T. Huang, K.-N. Ting and K.-L. Wang, Anal. Chim. Acta, 2008, 620, 120–126 CrossRef CAS PubMed.
- S. Ando and K. Koide, J. Am. Chem. Soc., 2011, 133, 2556–2566 CrossRef CAS PubMed.
- F. Song, S. Watanabe, P. E. Floreancig and K. Koide, J. Am. Chem. Soc., 2008, 130, 16460–16461 CrossRef CAS PubMed.
- D. H. Kwan, H. M. Chen, K. Ratananikom, S. M. Hancock, Y. Watanabe, P. T. Kongsaeree, A. L. Samuels and S. G. Withers, Angew. Chem., 2011, 123, 314–317 CrossRef.
- A. W. Czarnik, Chem. Biol., 1995, 2, 423–428 CrossRef CAS PubMed.
- S.-T. Huang, Y.-X. Peng and K.-L. Wang, Biosens. Bioelectron., 2008, 23, 1793–1798 CrossRef CAS PubMed.
- M. Sánchez-Martínez, M. Aguilar-Caballos and A. Gómez-Hens, J. Pharm. Biomed. Anal., 2004, 34, 1021–1027 CrossRef PubMed.
- Q.-J. Ma, X.-B. Zhang, X.-H. Zhao, Z. Jin, G.-J. Mao, G.-L. Shen and R.-Q. Yu, Anal. Chim. Acta, 2010, 663, 85–90 CrossRef CAS PubMed.
- W. Lin, X. Cao, Y. Ding, L. Yuan and Q. Yu, Org. Biomol. Chem., 2010, 8, 3618–3620 Search PubMed.
- M. H. Lee, S. W. Lee, S. H. Kim, C. Kang and J. S. Kim, Org. Lett., 2009, 11, 2101–2104 CrossRef CAS PubMed.
Footnote |
† Electronic supplementary information (ESI) available. See DOI: 10.1039/c6ra11735j |
|
This journal is © The Royal Society of Chemistry 2016 |
Click here to see how this site uses Cookies. View our privacy policy here.