DOI:
10.1039/C6RA13185A
(Paper)
RSC Adv., 2016,
6, 64769-64777
Acetylcholinesterase (AChE)-mediated immobilization of silver nanoparticles for the detection of organophosphorus pesticides†
Received
21st May 2016
, Accepted 2nd July 2016
First published on 4th July 2016
Abstract
In this work, we report an enzyme-mediated biosensor with the immobilization of silver nanoparticles (AgNPs) for the detection of organophosphorus (OP) pesticides. Acetylcholinesterase (AChE) catalyzes the hydrolysis of acetylthiocholine into thiocholine, which has a thiol molecule that inhibits the growth of AgNPs. The developed method is based on the inhibition of the enzyme by malathion or trichlorfon, which blocks the generation of thiocholine and leads to the growth of AgNPs during immobilization. The efficiency of enzyme activity was determined during the addition of OP pesticides, which can be correlated to the increase in intensity of the AgNP peak at 420 nm. The increase in intensity of AgNPs was dependent on the concentrations of OPs, and thus, this method allowed low-level detection of 0.455 nM and 5.46 nM for malathion and trichlorfon, respectively, by UV-visible spectroscopy. Further, the proposed detection method was successfully utilized for the detection of OP pesticides in different spiked real samples.
1. Introduction
Organophosphorus (OP) pesticides such as diazinon, parathion, paraoxon etc. are widely used in modern agriculture to protect crops from pests and insects.1 On the other hand, these pesticides are highly toxic substances, which can cause adverse effects to human health by affecting the central nervous system, resulting in neuromuscular malfunctions that could lead to death. The enzyme, acetylcholinesterase (AChE), is mainly found in cholinergic neurons and can catalyze the rapid hydrolysis of acetylcholine into choline and acetate. The enzyme AChE is inhibited by many chemical compounds, which include toxins like nerve gases or pesticides and drugs. Organophosphorus, organochlorine, or carbamate pesticides can cause acetylcholine accretion and lead to convulsions and suppression of breathing in human beings.2 The inhibition of AChE activity by OP pesticides results in a decrease in AChE signal inversely proportional to the concentration of OP pesticides, and thus, this reaction has been used for the detection of OP pesticides in combination with nanoparticles to improve the sensitivity.
The practical use of noble metal nanoparticles (NPs) for biological and biomedical applications are widely explored due to their strong surface plasmon resonance (SPR).3,4 The metallic NPs possess unique physical, chemical, and biological properties due to their nanometer-size range and shape.5,6 The unique optical properties of gold (Au) and silver (Ag) NPs result from their strong absorption and scattering of light.7 Among other NPs, AgNPs have been utilized in surface enhance Raman scattering (SERS), plasmonic absorbance, catalysis, antimicrobials, and as biosensors.8,9 Due to their biocompatibility, AgNPs are widely exploited in combination with biologically active molecules that can impart response to toxic chemical agents.10 Several studies have been reported for the rapid and direct detection of AChE inhibitors in aqueous solutions by simple and sensitive colorimetric AChE-based assays using NPs,11 of which AuNPs and AgNPs are widely used for OP pesticide detection in environmental and biological systems due to their distinct colorimetric properties.9,12 Therefore, AgNPs have been receiving great interest in recent years in the field of novel biosensors and as a very efficient support matrix for enzyme immobilization.13,14 Enzyme immobilization on NPs can be done to lower protein unfolding, which increases the enzyme stability (and activity) and biosensor performance.15
Thus, to improve the sensitivity for OP pesticide detection, recent studies report the immobilization of AChE on NPs. Ganesana et al. have developed a highly sensitive enzyme-based electrochemical biosensor for the detection of OP pesticide (paraoxon) by immobilization of (His)6-tagged AChE on nickel NPs with a detection limit of 0.001 nM.16 Similarly, Won et al. have performed immobilization of AChE on biomagnetic nanocomposites, consisting of iron oxide NPs (core)/silica (shell) for the detection of paraoxon with a detection limit of 5 nM.17 However, these developed methods pose complications with regard to the preparation or modification of working electrode, biosensor fabrication, enzyme immobilization process, and also requires more time. Colorimetric detection methods facilitate the performance of surface-immobilized enzyme assay on glass supports as compared to electrochemical detection method. Pavlov et al. used amino-functionalized AuNP seed-coated glass surface to determine the inhibitory constant of 1,5-bis(4-allyldimethylammonium-phenyl)pentan-3-one dibromide in the presence of AChE and ATCh.18 It was observed that as the concentration of inhibitor increases, the growth of AuNPs on the glass slides was also inhibited, which in turn tend to decrease their intensities at λ = 570 nm. Similarly, Virel et al. substantiated that the generation of thiocholine blocks the growth of Au–AgNPs during the formation of NPs in the absence of inhibitor.19 Further, to confirm the effect of thiol group binding on NPs, a control experiment was also performed in the presence of thiol group-containing, β-mercaptoethanol, and there was no absorption peak noted at λ = 400 nm, and thus, the generation or presence of thiol group hinders the growth of NP formation. Although, most of these methods allowed low detection limits and high sensitivity, these methods require growth/seed solutions for the formation and immobilization of NPs on a solid support and lacked any suggestion for on-site analysis.
In our previous work, we have described the detection of OP pesticides using an ex situ synthesis and modulated synthesis of AgNPs in the presence of AChE and ATCh.20,21 The developed method for the detection of OP pesticides using colloidal AgNPs influences the aggregation of NPs in aqueous phase, and the leaching of NPs tend to cause adverse effect to aquatic life due to long-term exposure.22–24 To overcome this problem, immobilization of AgNPs on a solid support is the most beneficial approach to control the release of NPs to the environment. Therefore, it is essential to develop an enzyme-immobilized NP sensor for the detection of OP pesticides in aqueous solutions and real samples.
In the present work, we report an enzyme-based biosensor for the estimation of both malathion and trichlorfon (OP pesticides) using enzyme immobilized on AgNPs. To the best of our knowledge, this is the first-ever study based on the enzyme immobilization of AgNPs on glass surface for achieving low-limit detection of OP pesticides (malathion and trichlorfon). This work provides a simple, biocompatible, and portable system with a rapid response time and can be extended for on-site analysis of OP pesticides. Enzyme immobilization increases the enzymatic activity and stability and yields high binding capacity using a glass support and also increases the stability of AgNPs in the immobilized condition. The detection was accomplished by immobilizing an amino-functionalized glass substrate using 3-aminopropyltriethoxysilane (APTES), with AgNPs in the presence of AChE and ATCh. The assay system is mainly based on the immobilization of the enzymatically yielded product, TCh, which blocks the immobilization of AgNPs in the absence of an OP inhibitor, and thereby, leads to decrease in the AgNP absorbance peak at 420 nm. Consequently, when the enzyme is inhibited in the presence of an inhibitor, the yield of thiol molecule greatly decreases. As the concentration of OP pesticides increases, the absorbance peak at 420 nm also increases. The proposed method demonstrated a high sensitivity with the lowest detection limit of 0.455 nM and 5.46 nM for malathion and trichlorfon, respectively. The developed method showed excellent potential and desired procedural simplicity for monitoring traces of malathion and trichlorfon in groundwater, lake water, and agricultural residue water samples.
2. Experimental section
2.1. Materials and chemicals
Acetylcholinesterase (AChE, from Electrophorus electricus) and 3-aminopropyl triethoxysilane (99%) were obtained from Sigma-Aldrich, India. Analytical grade pesticides, malathion (98.7%) and trichlorfon (96.7%), were purchased from Sigma-Aldrich, India. Trisodium citrate dihydrate (Na3C6H5O7·2H2O), sodium borohydride (NaBH4), and silver nitrate (AgNO3) were procured from SRL Pvt. Ltd (India). Glass slides (plain, dimension of 25.4 mm × 76.2 mm × 1 mm), tris(hydroxymethyl)aminomethane (tris buffer), and acetylthiocholine iodide (ATChI) were obtained from Himedia Laboratories Pvt. Ltd (India). Hydrogen peroxide (30%), sulphuric acid (98%), methanol (99%), and ethanol (99.9%) were purchased from SD Fine Chemicals Ltd (India).
The stock solutions of malathion (10−3 M) were prepared freshly in ethanol, and further, the solutions were diluted with deionized water (Milli-Q) to the appropriate dilution for further experimental use. However, trichlorfon (10−3 M) was prepared in Milli-Q. AChE (100 mU mL−1) and ATChI (100 mM) stock solutions were prepared using tris buffer solution (10 mM pH 7.4) and stored in the refrigerator at 4–5 °C when not in use. The iodide ions in ATChI solution was removed by our previous method to enhance the limit of detection.25
2.2. Instrumentation
UV-visible spectroscopic studies were performed using the diffuse reflectance measurement (DRS) method using a UV-visible absorption spectrometer (UV-2600, Shimadzu, Tokyo, Japan), and all the measurements were made in the spectral range from 200 to 800 nm. The topographical features of immobilized AgNPs were measured by Atomic Force Microscopy (AFM, Nanosurf easyScan2, Switzerland). The AFM measurements were performed in contact mode.
2.3. Preparation of colloidal silver nanoparticles
Silver nanoparticles were synthesized using a chemical reduction approach using sodium borohydride (NaBH4) as the reducing agent and trisodium citrate (TSC) as the stabilizing agent (also a mild reducing agent). For the immobilization of AgNPs on a glass substrate, the synthesis method was slightly modified by using Lee–Meisel method.26 Silver nitrate (AgNO3) was used as a precursor for AgNP synthesis, and the ratio of precursor, reducing agent, and stabilizing agent was maintained as 1% of AgNO3, 0.1% of NaBH4, and 1% of TSC. Briefly, AgNPs were prepared by adding 5 mL of TSC (1%) to 50 mL of Milli-Q water under vigorous stirring, followed by the addition of 0.5 mL of AgNO3 (1%) to the aqueous solution. Finally, 0.6 mL of NaBH4 (0.1%) was added to the solution, and a sudden color change was observed from colorless to a yellow color. Further, the resulting solution was allowed to stir for 1 h. The synthesis steps were performed in dark conditions, and the AgNPs were allowed to stabilize by avoiding any disturbance for 24 h at room temperature (27 ± 2 °C). The formation of AgNPs was confirmed by the strong surface plasmon band at 420 nm in the UV-visible spectrum (for detail see in ESI Fig. S1†).
2.4. Glass substrate preparation
Glass slides were used as the substrate for surface-immobilization of AChE by using the following method, which has been optimized for the uniform immobilization of enzyme on AgNPs.18,27 The residual oxide layer on the glass surface was cleaned with piranha solution (3
:
1, conc. H2SO4 – 30% H2O2) for 1 hour before functionalization of the glass substrate. After the cleaning of glass slides with Milli-Q several times, the slides were rinsed with ethanol and dried in an oven at 60 °C.
2.5. Immobilization of AChE and ATCh on glass substrate
After cleaning, the silanization process was carried out for the immobilization of AChE and ATCh on the glass substrate. The glass surface can be modified by using 3-aminopropyl triethoxysilane (APTES), which has been widely used for the immobilization of AgNPs on glass surfaces, as the amino groups (–NH2) in aminosilanes promote the adhesion on glass.28,29 The silanized glass substrates were immersed in a solution containing 10% of APTES prepared using absolute ethanol (solvent) and incubated for 20 min at room temperature (RT). Afterward, the amine-functionalized glass slides were removed and rinsed with methanol and Milli-Q, and then, dried in an oven at 60 °C. The amine-functionalized glass substrate was immersed for 20 min in 10 mM of tris buffer solution (pH 7.4 ± 0.2) containing a mixture of preincubated AChE (0.1 mU mL−1) and ATCh (1 mM) (preincubation time 20 min) in the absence of inhibitor. Scheme 1 demonstrates the immobilization of enzyme on a glass surface using an amine-functionalized glass substrate.
 |
| Scheme 1 Schematic representation of the procedure for enzyme immobilization on an amine-functionalized glass substrate. | |
2.6. OP pesticide detection using AChE immobilization
The inhibition of enzyme was studied by using two different OP pesticides such as malathion and trichlorfon. Different concentrations of malathion from 1 to 100 nM and trichlorfon from 5 to 100 nM were prepared in separate Coplin jars. The stock solutions of both pesticides were prepared separately using 10 mM of tris buffer (pH 7.4 ± 0.2). The amine-functionalized glass slides were immersed in the preincubated solution containing a mixture of AChE (0.1 mU mL−1) and ATCh (1 mM) for 20 min. The glass substrates were removed and immersed in solutions containing different concentrations of malathion (1 to 100 nM) and trichlorfon (5 to 100 nM) and incubated separately for 20 min at RT. Then, the glass substrates were removed from the OP pesticide solution and immediately immersed in the colloidal AgNP solution for 30 min at RT. Following this, the slides were washed with Milli-Q water to remove loosely bound AgNPs on the glass surface, and their absorbance was recorded at 420 nm using UV-visible spectroscopy, and their calibration curve was plotted between absorbance versus different concentrations of OP pesticides.
2.7. OP pesticide detection in real samples
In order to measure the OP pesticides in the environment, existing real samples like ground water, lake water, and agricultural residue water were analyzed by using the developed method. The ground water was collected from the near locality of Vellore. The agricultural residue water was obtained from the paddy field (Vellore, India). The lake water was collected from the VIT University Lake (Vellore, India). The collected samples were filtered through a Whatman filter paper and then through a nylon filter (0.22 μm) to remove any unwanted residue materials. All the water samples were stored at 4 °C when not in use, and the samples were used as stock solutions. Two different concentrations (10 and 80 nM) of both malathion and trichlorfon pesticides were spiked separately in ground water, lake water, and agricultural residue water samples, respectively. The control samples without spiking of OP pesticides were also prepared from the stock solutions.
3. Results and discussion
3.1. Reaction scheme
Scheme 2 represents the principle behind the mechanism for the detection of OP pesticide using an enzyme-mediated immobilization of AgNPs. During enzyme immobilization process, the resistance of the enzyme increases against environmental changes like temperature and pH, and also provides higher shelf life for the immobilized enzyme.30 The enzymatic reaction mixture (AChE and ATCh) was immobilized on the amine-functionalized glass substrate, and the enzyme hydrolyzes its substrate ATCh into TCh during enzyme immobilization. This enzyme-immobilized glass substrate when immersed in AgNP solution, the growth or enlargement of AgNPs gets hindered by the thiol groups present on TCh. As the production of TCh increases in the absence of inhibitors, the growth or enlargement of AgNPs gets blocked and results in the decrease in absorbance intensity of AgNPs at 420 nm. When the enzyme-immobilized glass substrate was immersed in OP pesticide solution before immersion in AgNP solution, an increase in the absorbance intensity of AgNPs was observed at 420 nm. When AChE was inhibited by the OP pesticide, the production of TCh molecule reduces, and consequently, the electrostatic repulsive force between the citrate ions on the surface of AgNPs increases,31 leading to an increase in the immobilization of AgNPs with a corresponding increase in the absorbance intensity at 420 nm. Therefore, the immobilization of AgNPs would be directly proportional to the concentration of OP pesticides.
 |
| Scheme 2 Graphical representation of enzyme immobilization for the detection of organophosphorus pesticide using AgNPs. | |
3.2. Optimization conditions
Initially, the control experiments were performed to confirm the obtained increase or decrease in UV-visible absorbance signal after the immobilization of AgNPs was done. For this, the UV-visible spectra for glass slides immobilized in APTES (10%) alone were recorded, and no increase in absorbance signal was noted from 200 to 800 nm (for detail see ESI Fig. S2†). Similarly, the amine-functionalized glass substrates were immersed in a solution containing a mixture of AChE (0.1 mU mL−1) and ATCh (1 mM) in the absence and presence of malathion (1 μM) separately for 20 min, and their absorbance spectra were recorded (shown in ESI Fig. S2†). As shown in Fig. S2,† there was no increase in absorbance intensity. Thus, the control experiment revealed that the increase in absorbance signal could be achieved by the immobilization of AgNPs only.
Further, the increase in signal intensity during the immobilization of AgNPs alone (without AChE and ATCh) was optimized by varying the interval time to obtain uniform immobilization of AgNPs on the amine-functionalized glass substrate. The amine-functionalized glass substrate was immersed in colloidal AgNPs solution for different interval times from 0 to 60 min. As the time increases during the immobilization of AgNPs, a gradual increase in the absorbance intensity of AgNPs was observed at 420 nm (for detail see in ESI Fig. S3†). Fig. S3† revealed that as the AgNP immobilization time increases, the absorbance intensity measured for the glass slides also increased up to 30 min and further increase in time led to gradual decrease in the AgNP absorbance intensity. From Fig. S3,† the maximum immobilization of AgNPs was found to be achieved within 30 min, and thus, this optimum time for dipping the enzyme-immobilized glass substrate in the AgNP solution was preferred throughout the experiment.
In order to carry out uniform immobilization of enzymatic mixture on the glass substrate, minimal concentrations of both AChE and ATCh are required. Hence, an experiment was carried out by varying the concentrations of AChE and ATCh on the amine-functionalized glass substrate, and then, the enzyme-immobilized glass substrates were immersed in AgNP solution, and the changes in absorbance intensities were recorded. The influence of concentration of enzyme substrate (ATCh) was studied by varying the ATCh concentrations from 0.25 to 2 mM in the presence of a fixed concentration of AChE (0.1 mU mL−1) as shown in Fig. 1A. As the concentration of ATCh increases, excess of thiol molecules will be generated, and this prevented the growth and immobilization of AgNPs on the enzyme-immobilized substrate, and a decrease in absorbance intensities of the AgNP peak at 420 nm was noted (Fig. 1A). From the linear graph, Fig. 1B, the ATCh concentration (1 mM) was chosen to obtain maximum production of TCh molecule. Similarly, the AChE concentration was determined by varying the concentrations of AChE from 0.02 to 1.0 mU mL−1 in the presence of fixed ATCh concentration (1 mM) as shown in Fig. 2A. When the AChE concentration increased, the system showed a maximum decrease in absorbance for 1.0 mU mL−1 of AChE concentration. From the calibration curve, Fig. 2B, the concentration of AChE (0.1 mU mL−1) was obtained, and this concentration was used to obtain maximum enzymatic activity with 1 mM of ATCh concentration.
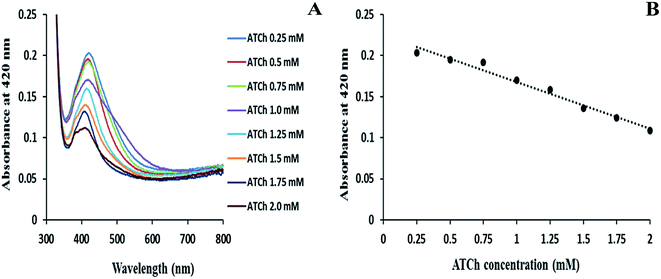 |
| Fig. 1 (A) Absorbance spectra of AgNPs immobilized on the glass surface in the presence of fixed AChE concentration (0.1 mU mL−1) with different concentrations of ATCh (0.25 to 2 mM). (B) Calibration curve for the variation of the absorbance peak (λ = 420 nm) at different ATCh concentrations. | |
 |
| Fig. 2 (A) Absorbance spectra of AgNPs immobilized on the glass surface in the presence of fixed ATCh concentration (1 mM) with different concentrations of AChE (0.02 to 1 mU mL−1). (B) Calibration curve for the variation of the absorbance peak (λ = 420 nm) at different AChE concentrations. | |
The influence of reaction time for the interaction of OP analytes with the enzyme-immobilized substrate was also studied. For determining the reaction time for AChE inhibition, the effect of a fixed concentration of malathion (1 μM) on the absorbance spectra of AgNPs at 420 nm was recorded by varying the pesticide interaction time from 5 to 60 min. The enzyme-immobilized glass substrates (AChE and ATCh) were immersed in malathion solutions for different intervals of time from 5 to 60 min (for detail see ESI Fig. S4†). Then, the each glass substrate was removed from the malathion solution and immersed in AgNP solution for 30 min. After the slides were washed with Milli-Q water, their absorbance was recorded at 420 nm. As the enzyme inhibition time increases from 5 to 30 min, an increase in the AgNP absorbance was observed. Further increase in time beyond 30 min yielded a gradual decrease in the absorbance probably due to the decrease in enzyme activity, and later, the enzyme activity reached a saturation point at 60 min (Fig. S4†). According to the calibration curve, the maximum inhibition of AChE activity was observed at 20 min. Thus, the optimal inhibition time (20 min) was chosen for further analysis using the AChE inhibition assay.
The effect of pH on the enzymatic mixture solution (AChE and ATCh) was observed in the absence and presence of OP pesticide solution, before and after immobilization on glass substrate. The pH of AgNP solution before and after AgNP immobilization on glass substrate was found to range between from 6.5 to 7.5. There were no apparent pH changes observed throughout the experiment, and hence, no adjustments were made to the experimental pH of the solution.
Further, the experiments was carried to determine the amount of substrate (acetylthiocholine) hydrolyzed by acetylcholinesterase (AChE) using Ellman's method.32 The Ellman's method was carried out for suspension and immobilized conditions, and the obtained results were compared between both the conditions (for detail see ESI Table S1†). As per the present immobilized system, the Ellman's method was performed with the same parameters (i.e. incubation time, concentration of AChE (0.1 mU), and ATCh (1 mM)) for both suspension and immobilized conditions. The AChE activity is estimated by the yellow color produced by the reaction between thiocholine and 5,5′-dithiobis-2-nitrobenzoic acid (DTNB). The production of yellow color due to the formation of 5-thio-2-nitro-benzoic acid caused a change in absorbance, which can be measured at 412 nm. The substrate (TCh) hydrolyzed per minute was calculated from the values obtained from suspension and immobilized conditions (Table 1). The obtained results from Table S1† reveals that the enzyme activity of AChE to produce thiol in the suspension condition is lower as compared with the activity observed on enzyme-immobilized glass support under the optimized conditions.
Table 1 Comparison of OP detection limits using enzyme immobilization with nanoparticles
Nanoparticles/substrate |
Method |
Pesticide |
LOD (nM) |
Ref. |
Nickel |
Electrochemical |
Paraoxon |
0.001 |
16 |
Iron oxide |
Electrochemical |
Paraoxon |
5 |
17 |
Mesoporous silica thin films |
Electrochemical |
Dichlorvos |
3.1 |
34 |
AgNPs |
Colorimetric |
Malathion |
0.455 |
Present work |
Trichlorfon |
5.46 |
3.3. Characterization studies
The stability of as-synthesized AgNPs in the colloidal solution was characterized by UV-visible spectroscopy. The formation of AgNPs exhibits a yellow color, and their spectrum was recorded at 420 nm (Fig. S1†). The topographical morphology of the enzyme-immobilized slides containing AgNPs were characterized using AFM, and the slides were analyzed both in absence and presence of OP pesticide. From Fig. 3, the 2D AFM images of AgNP arrays resulting from the immobilization of AgNPs in the absence (Fig. 3A) and the presence of 10 nM of malathion (Fig. 3C) can be observed. Further, the AFM 3D image affirmed that the size distribution of AgNPs on glass surface (Fig. 3B) in the absence of malathion was less uniform than that observed in the presence of malathion (Fig. 3D). The AFM image supports our understanding that the immobilization of AgNPs increases in the presence of enzyme inhibitor, which hinders the production of thiol-containing TCh during the hydrolysis of ATCh by AChE. The size of enzyme-immobilized AgNPs on the glass surface obtained from AFM images was observed to be larger or aggregated due to the AFM tip convolution, which may yield misleading information when the cross-sectional views (Fig. 3A and B) of the samples alone were considered.33
 |
| Fig. 3 AFM images of AgNPs coated on enzyme-immobilized glass surface in absence of malathion (A) 2D image and (B) 3D image, and in presence of 10 nM of malathion (C) 2D image and (D) 3D image. | |
3.4. Detection of OP pesticides using enzyme-immobilized substrate
Using the optimized concentrations of AChE (0.1 mu mL−1) and ATCh (1 mM), the enzyme immobilization of AgNPs was performed to determine the concentration of OP pesticides. The enzyme-immobilized glass substrates were immersed in different concentrations of malathion for 20 min at RT. Fig. 4 depicts the variation in the absorbance spectra of enzyme-immobilized glass substrate when exposed to different concentrations of malathion (0 to 100 nM) solution. The enzyme-immobilized substrate in the absence of malathion shows a pale yellow color with a very low absorbance at 420 nm (for detail see ESI Fig. S5†). Thus, the low AgNP intensity in the absence of inhibitor confirmed that the generation of thiol group reduced the level of AgNP immobilization. As the concentration of malathion increased, the surface plasmon absorbance band for AgNPs also increased in their absorbance, and the glass surfaces displayed an intense yellow color (Fig. S5†). Fig. 4A showed that as the concentration of malathion increased, the absorbance at 420 nm also increases, and a slight shift in intensities towards the blue region was observed due to the variation in size and electron density of NPs during the immobilization of AgNPs.21 Increase in malathion concentration resulted in growth of AgNPs by hindering the enzyme activity. A calibration curve value with R2 = 0.9656 was obtained by plotting the absorbance of AgNPs at 420 nm versus different concentrations of malathion as shown in Fig. 4B. The detection limit (LOD) for malathion was found to be 0.455 nM with a signal-to-noise ratio of 3.
 |
| Fig. 4 (A) Absorbance spectra of AgNPs immobilized on glass supports using a preincubated mixture of ATCh (1 mM) and AChE (0.1 mU mL−1) and variable concentrations of malathion from 0 to 100 nM. (B) Calibration curve for the detection of malathion (λ = 420 nm). | |
Similarly, Fig. 5 demonstrated the detection of trichlorfon in the range, 5–100 nM, with the optimized conditions using AChE-immobilized glass substrates. The increase in concentration of trichlorfon tends to hinder the hydrolysis activity of AChE (conversion of ATCh → TCh), which consequently increases the absorbance intensity of AgNPs at 420 nm proportional to the trichlorfon concentration (Fig. 5A). The LOD for trichlorfon was calculated to be 5.46 nM by plotting a graph for the absorbance of AgNPs at 420 nm versus different concentrations of trichlorfon as shown in Fig. 5B, and a calibration curve with R2 = 0.9637 was obtained. The proposed method was able to detect OP pesticides using enzyme immobilization of AgNPs with higher sensitivity as compared with other earlier reported methods (Table 1).
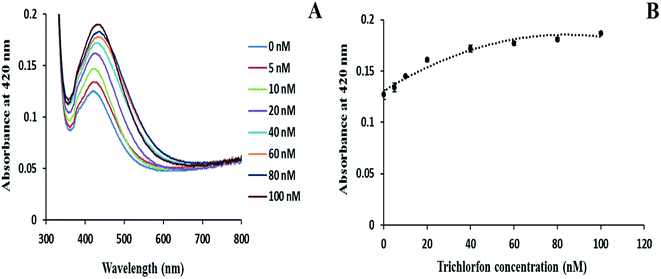 |
| Fig. 5 (A) Absorbance spectra of AgNPs immobilized on glass supports using a preincubated mixture of ATCh (1 mM) and AChE (0.1 mU mL−1) and variable concentrations of trichlorfon from 0 to 100 nM. (B) Calibration curve for the detection of trichlorfon (λ = 420 nm). | |
Statistical analysis was performed using one-way ANOVA test to analyze the statistical significance of the obtained results for both malathion and trichlorfon pesticides. Both the experiments were performed in triplicates, and the significance value was found to be less than 0.05 for both pesticides, which ensured the sensitivity of the system. The robustness of the present techniques were ascertained by performing run-to-run, day-to-day, and batch-to-batch tests for both malathion and trichlorfon, respectively (Table 2).
Table 2 Statistical analysis for detection of malathion and trichlorfon
Analyte |
Linear range (nM) |
LODa (nM) |
RSDb% (n = 3) |
Run-to-run |
Day-to-day |
Batch-to-batch |
Limit of detection. Relative standard deviation measured from 3 parallel experiments. |
Malathion |
1 to 100 |
0.455 |
5.2 |
4.1 |
2.1 |
Trichlorfon |
5 to 100 |
5.46 |
4.8 |
2.5 |
1.2 |
3.5. Real sample analysis for malathion and trichlorfon
The efficiency of the designed system to measure different concentrations of OP pesticides, namely malathion and trichlorfon, spiked in real samples (groundwater, lake water, and agricultural residue water) was analyzed to validate the potential application of the current method. The enzyme-immobilized glass substrates (AChE and ATCh) were immersed in two different concentrations (10 and 80 nM) of malathion- and trichlorfon-spiked water samples to determine the OP pesticide concentrations in groundwater, lake water, and agricultural residue water samples. The glass slides were allowed to incubate for 20 min at RT, and then, removed from the spiked samples and immersed in AgNP solution for 30 min. Finally, the glass slides were washed with Milli-Q water, and their concentrations were analyzed by measuring the absorbance of AgNPs using UV-visible spectroscopy. Tables 3 and 4 summarizes the recovery and standard deviation (RSD), and the obtained values were found to be satisfactory for the detection of malathion and trichlorfon in real samples. The values obtained from Tables 3 and 4 demonstrated good recovery for OP pesticides spiked in real samples.
Table 3 Detection of malathion in real samples
Sample |
Added (nM) |
Found (nM) |
Recovery (%) |
RSD (%) |
Groundwater |
10 |
9.4 |
94.00 |
3 |
80 |
73 |
91.25 |
0.7 |
Lake water |
10 |
8.4 |
84.00 |
1 |
80 |
76 |
95.00 |
0.4 |
Agricultural residue water |
10 |
9 |
90.00 |
1.5 |
80 |
75 |
93.75 |
1.1 |
Table 4 Detection of trichlorfon in real samples
Sample |
Added (nM) |
Found (nM) |
Recovery (%) |
RSD (%) |
Groundwater |
10 |
9.2 |
92.00 |
0.9 |
80 |
67.1 |
83.88 |
0.8 |
Lake water |
10 |
8.7 |
87.00 |
0.5 |
80 |
67.6 |
84.50 |
2.8 |
Agricultural residue water |
10 |
9.7 |
97.00 |
0.4 |
80 |
74.1 |
92.63 |
1.4 |
4. Conclusion
A simple, rapid, and sensitive method was developed by the immobilization of enzyme and AgNPs on a glass substrate, which was used as a sensing probe for the detection of OP pesticides. Since OP pesticides like malathion and trichlorfon are AChE inhibitors, the enzymatic activity of AChE considerably decreases in the presence of these inhibitors. In addition, the AgNP signal amplification was proportional to the growth of AgNPs during the immobilization process. The adopted immobilization procedure was simple, highly reproducible, and yielded the quantitative analysis of OP pesticides with minimum leaching of NPs, and it can be easily used for the on-site analysis using a portable spectrophotometer. Furthermore, the immobilization of enzyme provides more stability, and thus, helps to achieve low detection limits of 0.455 nM for malathion and 5.46 nM for trichlorfon.
The use of AgNPs strongly influences the enzyme immobilization support for pesticide detection due to their small size, high surface area, and biocompatibility. However, the method has its limitations as amine-functionalized glass substrates are required for the uniform enzyme immobilization of AgNPs, which is quite expensive and requires glass substrate preparation. The proposed sensing method can be extended for the detection of other AChE inhibitors using a similar strategy.
Acknowledgements
The authors would like to acknowledge the funding from the Defence Research & Development Organization (ERIP/ER/1103964/M/01/1485), Ministry of Defence Government of India. We also acknowledge the VIT University for providing the AFM facility.
References
- K. Zhang, Q. Mei, G. Guan, B. Liu, S. Wang and Z. Zhang, Anal. Chem., 2010, 82, 9579–9586 CrossRef CAS PubMed.
- Y. Miao, N. He and J.-J. Zhu, Chem. Rev., 2010, 110, 5216–5234 CrossRef CAS PubMed.
- A. J. Haes, S. Zou, G. C. Schatz and R. P. Van Duyne, J. Phys. Chem. B, 2004, 108, 109–116 CrossRef CAS.
- P. K. Jain, X. Huang, I. H. El-Sayed and M. A. El-Sayed, Plasmonics, 2007, 2, 107–118 CrossRef CAS.
- J. A. Creighton and D. G. Eadon, J. Chem. Soc., Faraday Trans., 1991, 87, 3881–3891 RSC.
- Y. Cao, R. Jin and C. A. Mirkin, J. Am. Chem. Soc., 2001, 123, 7961–7962 CrossRef CAS PubMed.
- D. Paramelle, A. Sadovoy, S. Gorelik, P. Free, J. Hobley and D. G. Fernig, Analyst, 2014, 139, 4855–4861 RSC.
- J. C. Trefry, J. L. Monahan, K. M. Weaver, A. J. Meyerhoefer, M. M. Markopolous, Z. S. Arnold, D. P. Wooley and I. E. Pavel, J. Am. Chem. Soc., 2010, 132, 10970–10972 CrossRef CAS PubMed.
- S. Agnihotri, S. Mukherji and S. Mukherji, RSC Adv., 2014, 4, 3974–3983 RSC.
- L. S. Nair and C. T. Laurencin, J. Biomed. Nanotechnol., 2007, 3, 301–316 CrossRef CAS.
- A. Mulchandani, W. Chen, P. Mulchandani, J. Wang and K. R. Rogers, Biosens. Bioelectron., 2001, 16, 225–230 CrossRef CAS PubMed.
- K. Saha, S. S. Agasti, C. Kim, X. Li and V. M. Rotello, Chem. Rev., 2012, 112, 2739–2779 CrossRef CAS PubMed.
- L. S. B. Upadhyay and N. Verma, Bioprocess Biosyst. Eng., 2014, 37, 2139–2148 CrossRef CAS PubMed.
- S. A. Ansari and Q. Husain, Biotechnol. Adv., 2012, 30, 512–523 CrossRef CAS PubMed.
- M. N. Gupta, M. Kaloti, M. Kapoor and K. Solanki, Artif. Cells, Blood Substitutes, Biotechnol., 2011, 39, 98–109 CrossRef CAS PubMed.
- M. Ganesana, G. Istarnboulie, J.-L. Marty, T. Noguer and S. Andreescu, Biosens. Bioelectron., 2011, 30, 43–48 CrossRef CAS PubMed.
- Y.-H. Won, H. S. Jang, S. M. Kim, E. Stach, M. Ganesana, S. Andreescu and L. A. Stanciu, Langmuir, 2009, 26, 4320–4326 CrossRef PubMed.
- V. Pavlov, Y. Xiao and I. Willner, Nano Lett., 2005, 5, 649–653 CrossRef CAS PubMed.
- A. Virel, L. Saa and V. Pavlov, Anal. Chem., 2008, 81, 268–272 CrossRef PubMed.
- D. N. Kumar, S. Alex, R. S. Kumar, N. Chandrasekaran and A. Mukherjee, Colloids Surf., A, 2015, 485, 111–117 CrossRef CAS.
- D. N. Kumar, A. Rajeshwari, S. Alex, M. Sahu, A. Raichur, N. Chandrasekaran and A. Mukherjee, RSC Adv., 2015, 5, 61998–62006 RSC.
- B. De Gusseme, T. Hennebel, E. Christiaens, H. Saveyn, K. Verbeken, J. P. Fitts, N. Boon and W. Verstraete, Water Res., 2011, 45, 1856–1864 CrossRef CAS PubMed.
- S. Agnihotri, S. Mukherji and S. Mukherji, Nanoscale, 2013, 5, 7328–7340 RSC.
- A. Gupta and S. Silver, Nat. Biotechnol., 1998, 16, 888 CrossRef CAS PubMed.
- D. N. Kumar, A. Rajeshwari, S. A. Alex, N. Chandrasekaran and A. Mukherjee, New J. Chem., 2015, 39, 1172–1178 RSC.
- Y. Wan, Z. Guo, X. Jiang, K. Fang, X. Lu, Y. Zhang and N. Gu, J. Colloid Interface Sci., 2013, 394, 263–268 CrossRef CAS PubMed.
- Y. Jin, X. Kang, Y. Song, B. Zhang, G. Cheng and S. Dong, Anal. Chem., 2001, 73, 2843–2849 CrossRef CAS PubMed.
- K. Wu, Y. Chang, W. Tsai, M. Huang and C. Yang, Polym. Degrad. Stab., 2010, 95, 2328–2333 CrossRef CAS.
- P. Pallavicini, A. Taglietti, G. Dacarro, Y. A. Diaz-Fernandez, M. Galli, P. Grisoli, M. Patrini, G. S. De Magistris and R. Zanoni, J. Colloid Interface Sci., 2010, 350, 110–116 CrossRef CAS PubMed.
- A. Liese and L. Hilterhaus, Chem. Soc. Rev., 2013, 42, 6236–6249 RSC.
- Z. Li, Y. Wang, Y. Ni and S. Kokot, Sens. Actuators, B, 2014, 193, 205–211 CrossRef CAS.
- G. L. Ellman, K. D. Courtney, V. Andres and R. M. Featherstone, Biochem. Pharmacol., 1961, 7, 88–95 CrossRef CAS PubMed.
- J. C. Hulteen, D. A. Treichel, M. T. Smith, M. L. Duval, T. R. Jensen and R. P. Van Duyne, J. Phys. Chem. B, 1999, 103, 3854–3863 CrossRef CAS.
- X. Zhang, H. Jia, X. Wang, H. Zhang, H. Yin, S. Chang, J. Wang and W. Wu, Chin. Sci. Bull., 2009, 54, 3023–3028 CrossRef CAS.
Footnote |
† Electronic supplementary information (ESI) available. See DOI: 10.1039/c6ra13185a |
|
This journal is © The Royal Society of Chemistry 2016 |
Click here to see how this site uses Cookies. View our privacy policy here.