Tumor-targeting, enzyme-activated nanoparticles for simultaneous cancer diagnosis and photodynamic therapy†
Received
1st October 2015
, Accepted 12th November 2015
First published on 16th November 2015
Abstract
Specific targeting towards tumors and the on-site activation of photosensitizers to diagnose tumors and reduce side effects for patients are currently the main challenges for photodynamic therapy (PDT) in the clinic. Herein, uniform diiodostyryl bodipy conjugated hyaluronic acid nanoparticles (DBHA-NPs) were successfully synthesized. The evaluation of their PDT effect at both a cellular level and in animal models of tumor-bearing mice shows that the DBHA-NPs present a remarkable suppression of tumorous growth due to their specific targeting and enhanced permeability and retention (EPR) effect. More importantly, the enzyme-activated “self-assembly and disaggregation” behavior in tumors can lead to the on-site activation of DBHA-NPs, which can diagnose the tumor exactly and reduce the side effects for patients significantly. These findings confirm that DBHA-NPs have significant potential for photodynamically activated cancer theranostics in a clinical setting.
1. Introduction
Photodynamic therapy (PDT) is an effective therapeutic modality for a variety of malignant and nonmalignant tumors.1–4 Mediated by photosensitizers (PSs), cancer cells can be killed by reactive oxygen species (ROS), which are generated by locally sensitizing oxygen upon light irradiation.5 As for cancer treatment, PDT has emerged as an important method in preclinical research and clinical practice because of its non-invasive nature and selective light irradiation at the tumor site, avoiding damage to normal tissues and organs.
However, there are still several problems which prevent the development of PDT in the clinic. Firstly, traditional PSs suffer from their native hydrophobicity and poor biocompatibility, limiting their potential application in biological fields.6 At present, two main strategies have been developed to increase their water solubility and biocompatibility. In one approach, PSs can be conjugated with hydrophilic molecules through covalent bonds.7 In another approach, PSs can be incorporated into biocompatible drug delivery platforms via noncovalent bonds, such as in liposomes,8–11 polymeric micelles,12–14 and inorganic nanoparticles.15–20 Secondly, the ability to enhance the selectivity of highly effective PSs so that they can locate and function at the tumor site preferentially is another major challenge. A promising method is to directly conjugate PSs with targeting ligands, including folates, antibodies, proteins, and so on.21–23 Finally, the third obstacle for PDT in the clinic is that the activation of PSs at non-tumor sites would damage the normal tissues and organs of patients through blood circulation. Recently, activatable photosensitizing systems have drawn a lot of attention; these can be activated selectively by tumor-associated stimuli. Commonly, activatable PSs are photodynamically inactive in normal tissue environments and their fluorescence and ROS generation are silent even with light irradiation. However, these PSs become photodynamically active at tumor sites through various kinds of activation mechanisms, including enzymatic activation,24,25 nucleic acid-activation26,27 and tumor environment activation28 (e.g., the remarkable decrease in pH value and significantly increased level of H2O2). The activatable PSs can achieve excellent treatment selectivity and destroy targeted tumor cells, but not threaten normal tissues and organs.29 Therefore, the development of new kinds of PSs to solve all the problems mentioned above in PDT clinical use is extremely important.
In this study, diiodostyryl bodipy conjugated hyaluronic acid nanoparticles (DBHA-NPs) are designed for activatable photodynamic theranostics in tumors. The DBHA-NPs exhibit excellent hydrophilicity and biocompatibility, as well as specific targeting towards CD44 receptors overexpressed on the surface of tumor cells (e.g. HCT-116 cells, human colon carcinoma cell line). The DBHA-NPs reaching the lysosomes inside HCT-116 cells can be disaggregated, resulting in the restoration of fluorescence and ROS generation with light irradiation, which is impossible in the native diiodostyryl bodipy due to its aggregation (Fig. 1). Additionally, both in vitro and in vivo experiments demonstrate that DBHA-NPs can effectively label and photodynamically suppress the growth of tumors.
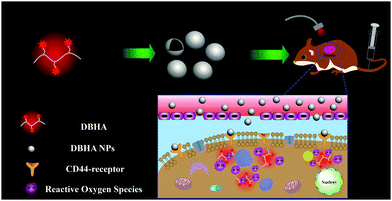 |
| Fig. 1 Schematic illustration of DBHA-NPs as theranostic agents for PDT both in vitro and in vivo. | |
2. Experimental section
Synthesis of DBHA-NPs
Fig. 2 shows the synthetic route of DBHA. In brief, 4-hydroxybenzaldehyde was treated with 3-bromo-1-propanol in the presence of K2CO3 in acetone to yield compound 1. Diiodo bodipy30,31 (compound 3) was prepared through the iodination of bodipy32,33 (compound 2), using N-iodosuccinimide (NIS) as the iodo-agent. Furthermore, compound 3 underwent Knoevenagel condensation with compound 1 to yield diiodostyryl bodipy (compound 4) through a microwave reaction.34 As is well known, diiodostyryl bodipy has great potential as the PS for PDT, being mainly disadvantaged by its native hydrophobicity and bad biocompatibility, as well as targeting.35–37 It can be concluded that the above diiodostyryl bodipy (compound 4) is further conjugated onto the hyaluronic acid (HA) through an esterification reaction. Notably, DBHA shows excellent water-solubility and good biocompatibility due to the existence of HA. Finally, DBHA dissolved in deionized (DI) water was added dropwise to a large amount of DI water under ultrasonication to yield DBHA-NPs through self-assembly.
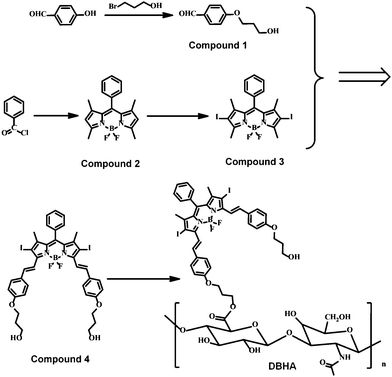 |
| Fig. 2 Synthetic route of DBHA. | |
Characterization
Nuclear magnetic resonance (NMR) spectra were obtained using a Bruker Ultra Shield Plus 400 MHz. GC-MS spectra were recorded using a Shimadzu GC-MS-QP 2010 Plus mass spectrometer. MALDI-TOF-MASS (matrix-assisted laser desorption/ionization time-of-flight mass spectrometry) was performed using a Bruker autoflex under reflector mode (without a matrix) for data acquisition. UV-visible absorption and photoluminescence (PL) spectra were recorded using a Shimadzu UV-3600 UV-VIS-NIR spectrophotometer and a RF-5301 PC spectrofluorophotometer, respectively. Transmission electron microscopy (TEM) images were obtained using a JEOL JEM-2100 transmission electron microscope. The hydrodynamic size of the nanoparticles (dynamic light scattering, DLS) was measured using a 90 Plus particle size analyzer (Brookhaven Instruments).
Cell lines and culture conditions
HCT-116 and A2780 cell lines, provided by the Institute of Biochemistry and Cell Biology, SIBS, CAS (China), were cultured in regular growth medium consisting of Dulbecco's modified Eagle's medium (DMEM, Gibco) supplemented with 10% fetal bovine serum at 37 °C under a 5% CO2 atmosphere. The cells were routinely harvested by treating with a trypsin–ethylene diamine tetraacetic acid (EDTA) solution (0.25%).
Cell viability assays
HA and DBHA-NPs were first dissolved in phosphate buffered saline (PBS) solution, then diluted with DMEM to various concentrations (0.2, 0.4, 0.6, 0.8, 1.0, 1.2, 1.4, and 1.6 mg ml−1). HCT-116 cells and A2780 cells, rinsed with PBS solution, were incubated with HA and DBHA-NPs solutions for 24 h at 37 °C. Then the cells were rinsed again with PBS solution and refilled with 100 μl of the culture medium before being illuminated at ambient temperature. The light source was a xenon lamp, equipped with a color glass filter with a cut-on at 600 nm. The fluence rate (λ > 600 nm) was 40 mW cm−2 for 7 minutes.
Cell viability was determined by means of the colorimetric MTT assay. An MTT (USB) solution in PBS solution (5 mg ml−1, 20 μl) was added to each well followed by incubation for 4 h under the same conditions at 37 °C. Then the medium was discarded and 150 μl of DMSO was added. The plate was agitated on a Bio-Tek microplate reader at ambient temperature before the absorbance at 490 nm for each well was measured. The average absorbance of the blank well (no cells) was subtracted from the readings of the other wells. The cell viability was then determined by the following equation: viability (%) = {∑[(Ai/Acontrol) × 100]}/n, where Ai is the absorbance of the ith data (i = 1, 2, 3,…,n), Acontrol is the average absorbance of the control wells in which HA or DBHA-NPs were absent, and n is the number of data points.
Flow cytometry study
Approximately 6 × 105 HCT-116 cells in the medium (2 ml) were seeded on a 6-well plate and incubated for 24 h under 5% CO2. The cells were treated with DBHA-NPs at various concentrations (0.25, 0.5, 0.75, and 1.0 mg ml−1) and incubated under the same conditions for 4 h. The cells were then rinsed thrice with PBS solution and refilled with 1 ml of the culture medium before being illuminated (λ > 600 nm, 40 mW cm−2, 7 min) at ambient temperature. After 24 h of incubation, the cell were rinsed with PBS solution and harvested by 0.25% trypsin (Invitrogen, 500 μl) for 2 min, followed by centrifugation at 1200 rpm. The cells were suspended in 100 μl of binding buffer containing FITC annexin-V (5 μl) and PI (5 μl). After incubation in darkness for 15 min at room temperature, the signals of FITC annexin-V and PI were measured using a BD FACSCalibur flow cytometer (Becton Dickinson) with 104 cells counted in each example. Both annexin-V and PI were excited by a 488 nm argon laser. The emitted fluorescence was monitored at 500–560 nm for annexin-V and at >670 nm for PI. The data collected were analyzed using Cell QUEST Pro.
Fluorescence microscopic study
About 2 × 105 HCT-116 cells or A2780 cells in the culture medium (2 ml) were seeded on a glass bottom Petri dish and incubated overnight at 37 °C. Then the medium was removed. The cells, rinsed with PBS solution, were incubated with a solution of DBHA-NPs (1 mg ml−1, 100 μl) in the medium for 4 h. The cells were rinsed with PBS solution and viewed with an Olympus IX 70 inverted microscope. The excitation light (588 nm) was provided by a multi-wavelength illuminator (Polychrome IV, TILL Photonics). The emitted fluorescence at >600 nm was collected, digitized, and analyzed using MetaFluor V6.3 (Universal Imaging). The average intracellular fluorescence intensities (24 cells for each sample) were determined.
Similarly, to confirm the concept of CD44 receptor mediated tumor cell endocytosis, about 2 × 105 HCT-116 cells in the culture medium (2 ml) were seeded on a glass bottom Petri dish and incubated overnight at 37 °C under 5% CO2. Then the medium was removed. Firstly, the cells were incubated with HA solution (2 mg ml−1, 100 μl) for 4 h, and further rinsed with PBS solution. Then the above cells were incubated with DBHA-NPs solution (1 mg ml−1, 100 μl) for 4 h under the same concentrations. The cells were rinsed with PBS solution and viewed with an Olympus IX 70 inverted microscope. The excitation light (588 nm) was provided by a multi-wavelength illuminator (Polychrome IV, TILL Photonics). The emitted fluorescence at >600 nm was collected, digitized, and analyzed using MetaFluor V6.3 (Universal Imaging).
Subcellular location study
About 1 × 105 HCT-116 cells in culture medium (2 ml) were seeded on a glass bottom Petri dish and incubated overnight at 37 °C under 5% CO2. The medium was removed and the cells were rinsed with PBS solution. The cells were incubated with DBHA-NPs solution in the medium (1 mg ml−1, 100 μl) for 24 h. For the study using Mito-Tracker, the cells were incubated with Mito-Tracker Green FM (Molecular Probes, 0.2 μM in the medium) for a further 15 min. For the study using Lyso-Tracker, green DND 26 (Molecular Probes, 0.075 μM in the medium) or ER-Tracker Green (Molecular Probes, 1.0 μM in PBS solution) for a further 15 min. For all the cases, the cells were rinsed with PBS solution and viewed with a Leica SP5 confocal microscope equipped with a 488 nm argon laser and a 588 nm helium neon laser. All trackers were excited at 488 nm and monitored at 510–560 nm, while DBHA-NPs were excited at 588 nm and monitored at 600–700 nm. The images were digitized and analyzed using Leica Application Suite Advanced Fluorescence software. The subcellular location of the DBHA-NPs was revealed by comparing the intracellular fluorescence images produced using the Mito-Tracker, Lyso-Tracker, ER-Tracker and DBHA-NPs.
Real-time monitoring of the fluorescence in vitro
About 1 × 105 HCT-116 cells in culture medium (2 ml) were seeded on a glass bottom Petri dish and incubated overnight at 37 °C under 5% CO2. The medium was removed, and the cells were rinsed with PBS solution, followed by the addition of a solution of DBHA-NPs (1 mg ml−1, 100 μl) into fresh medium for incubation at 37 °C. Meantime, the fluorescence of the DBHA-NPs was observed by Confocal Laser Scanning Microscope (CLSM) and the confocal images were obtained using a Zeiss confocal laser microscope (ZEISS LSM 700) at different times.
Fluorescence studies for ROS probe
About 2 × 105 HCT-116 cells in the culture medium (2 ml) were seeded on a glass bottom Petri dish and incubated overnight at 37 °C under 5% CO2. The medium was removed. The cells, rinsed with PBS solution, were incubated with a solution of DBHA-NPs or porphyrin in PBS solution (1 mg ml−1, 100 μl) for 24 h. The cells were rinsed with PBS solution and further incubated with the ROS probe, DCFH-DA (50 μg ml−1, 10 μl) for 1 h. Then, the cells were rinsed thrice with PBS solution and refilled with 1 ml of the culture medium before being illuminated at ambient temperature using a xenon lamp light (λ > 600 nm, 40 mW cm−2, 7 min). After that, the cells were viewed with an Olympus IX70 inverted microscope. The excitation light (488 nm) was provided by a multi-wavelength illuminator (Polychrome IV, TILL Photonics). The emitted fluorescence from 500 to 600 nm was collected, digitized, and analyzed using MetaFluor V6.3 (Universal Imaging).
For the comparison without cells, no cells were added and only DBHA-NPs or porphyrin with DCFH-DA at the same concentrations.
In vivo fluorescence and cancer treatment
All animal experiments were performed complying with the NIH guidelines for the care and use of laboratory animals. The School of Pharmaceutical Science, Nanjing Tech University (NanjingTech) approved the animal experiments. Xenograft tumors were established by subcutaneously injecting HCT-116 cells into the armpit of nude mice. After tumors of 30–40 mm3 were palpable, whole body images of the mice were acquired by a tail vein injection of 200 μl of DBHA-NPs at a concentration of 10 mg ml−1. All images were analyzed and collected at the indicated time points with a Maestro™ In-Vivo Imaging System (Cri, Woburn, MA, USA).
For the tumor treatment, the mice were randomly divided into four groups with six mice in each group. The mice in the first to the third group were injected with saline, HA and DBHA-NPs solution (10 mg ml−1, 200 μl) by a tail vein injection, with light irradiation (λ > 600 nm, 40 mW cm−2, 15 min). The mice in the fourth group were injected with DBHA-NPs solution (10 mg ml−1, 200 μl) by a tail vein injection without light irradiation while under the same conditions.
3. Results and discussion
Preparation and characterization of DBHA-NPs
Fig. 3a shows the 1H-NMR spectrum of DBHA in D2O. The appearance of peaks with chemical shifts between 7.0–8.0 in the spectra, consistent with those of HA38,39 suggests that HA has been successfully conjugated onto the diiodostyryl bodipy. The TEM image in Fig. 3b demonstrates that the self-assembled DBHA-NPs have a spherical morphology. The dynamic light scattering (DLS) measurement indicates the average size of the particles is around 50 nm (inset of Fig. 3b). Fig. 3c shows the optical absorption properties of DBHA, DBHA-NPs and HA dissolved in PBS. An obvious absorption peak at 600 nm arising from the Q-band of diiodostyryl bodipy can be observed. In contrast, there are no peaks for pure HA, indicating that diiodostyryl bodipy has been successfully conjugated onto the HA. Interestingly, after the addition of hyaluronidase which can degrade the HA polymer, the fluorescence of diiodostyryl bodipy (at 678 nm) recovers in a time-dependent manner (Fig. 3d and Fig. S1, ESI†), confirming the enzymatic degradation process of HA. With the degradation of HA, the DBHA NPs can also be de-aggregated and the fluorescence intensity increases.
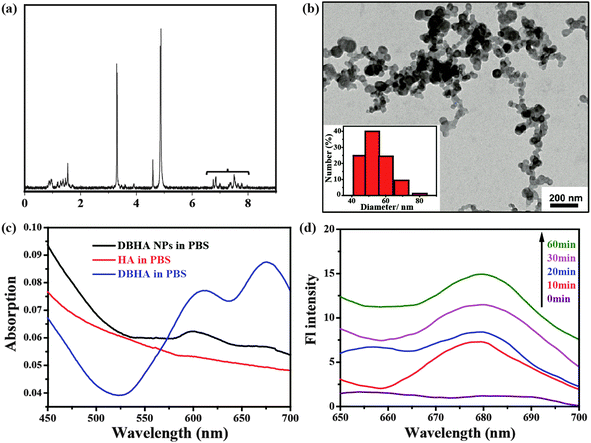 |
| Fig. 3 (a) 1H-NMR spectrum of DBHA in D2O. (b) TEM image of the DBHA-NPs. The inset shows the size distribution of the DBHA-NPs. (c) UV-Vis absorption of DBHA, DBHA-NPs and HA (5 mg ml−1) in PBS. (d) Fluorescence spectra of the DBHA-NPs (5 mg ml−1) incubated with hyaluronidase (0.1 mg ml−1) in PBS for different times, excited at 600 nm. | |
NIR fluorescence images and specific targeting of DBHA-NPs
CD44, a receptor for HA, is overexpressed in some types of cancer cells (e.g., HCT-116 cells, corresponding to colon cancer).40 As expected, Fig. 4a shows that the DBHA-NPs can abundantly stain the HCT-116 cells41 and are uniformly dispersed in the cytoplasm of the HCT-116 cells. In contrast, A2780 cells (human ovarian carcinoma cell line), known to have a low expression of CD44 receptors, are not well-stained by the DBHA-NPs and the fluorescence intensity is greatly decreased compared with that of the HCT-116 cells (Fig. 4b). It is well known that the staining can be eliminated by the previous addition of excess free HA molecules because of competitive inhibition. As shown in Fig. 4b, with the existence of HA, the fluorescence intensity of the HCT-116 cells decreases greatly, further indicating that the DBHA-NPs have specific targeting properties towards CD44 overexpressed HCT-116 cells. All the results suggest that DBHA-NPs are internalized via receptor mediated endocytosis triggered by the binding of DBHA-NPs with CD44 receptors.42 What’s more, the parallel filter evaluation indicates that the DBHA-NPs are mainly delivered into lysosomes, as shown in Fig. 4c and Fig. S2 (ESI†).
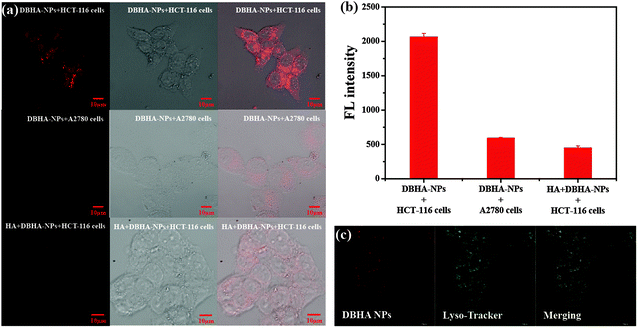 |
| Fig. 4 (a) Fluorescence images of DBHA-NPs in HCT-116 cells, A2780 cells and HA + DBHA-NPs in HCT-116 cells. The red color represents the fluorescence of the DBHA-NPs. (Left panel: fluorescence images. Middle panel: brightfield. Right panel: merged images.) (b) The intracellular fluorescence intensity of DBHA-NPs in HCT-116 cells, in A2780 cells and HA + DBHA-NPs in HCT-116 cells. All data were expressed as the mean ± standard deviation (number of experiments, n = 6). (c) Visualization of the intracellular fluorescence of HCT-116 cells using filter sets specific for DBHA-NPs (in red, column 1) and lysosome tracker (in green column 2). Column 3 shows the merged images in brightfield. | |
NIR fluorescent enzyme-activation of DBHA-NPs at tumorous sites both in vitro and in vivo
To investigate the on-site activation properties of the DBHA-NPs in tumors, in vitro and in vivo fluorescence images of DBHA-NPs entering the HCT-116 cells at different times are measured. Fig. 5a presents the fluorescence images of DBHA-NPs incubated with HCT-116 cells with time observed by confocal laser scanning microscopy (CLSM). It can be seen that the fluorescence becomes stronger and stronger with time from 0 to 60 minutes. A similar phenomenon has also been observed in vivo after the injection of DBHA-NPs in mice bearing HCT-116 cells, as shown in Fig. 5b. There is no obvious fluorescence observed before 8 h, even in the heart, liver, lung, brain and other organs and tissues.43–45 Strong fluorescence only appears at the tumor site after 24 h after administration of the DBHA-NPs. Both results indicate that DBHA-NPs only can be decomposed in cells or tumors, which makes them effective on-site activated PSs and beneficial for diagnosing the tumor site precisely. Furthermore, this result is also in good agreement with the previous characterization (Fig. 3d).
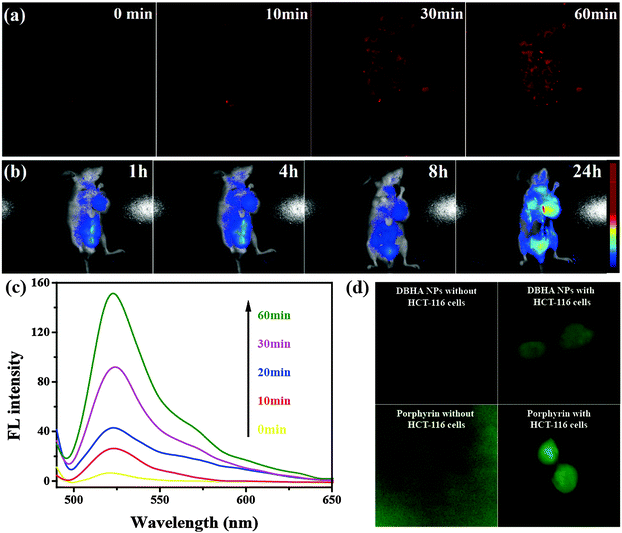 |
| Fig. 5 (a) Fluorescence images of DBHA-NPs entering HCT-116 cells observed by CLSM from 0 to 60 min. (b) In vivo fluorescence images of mice bearing HCT-116 cells in the armpit after tail-vein injection of DBHA-NPs. (c) Fluorescence intensity of the ROS probe after incubating with DBHA-NPs and hyaluronidase with light irradiation for different times. (d) Fluorescence images of DBHA-NPs and porphyrin with DCFH-DA out of or in HCT-116 cells upon light irradiation by CLSM. Column 1: DBHA NPs and porphyrin with DCFH-DA outside HCT-116 cells. Column 2: DBHA-NPs and porphyrin with DCFH-DA in HCT-116 cells. | |
Enzyme-activated generation of ROS
Reactive oxygen species (ROS) generated from PSs can cause oxidative stress and membrane damage in the treated cells and eventual cell death, which is an important factor for PDT. 2′,7′-Dichlorofluorescin diacetate (DCFH-DA), non-fluorescent in its native form, can react with ROS to produce fluorescent 2′,7′-dichlorofluorescein (DCF),46 which is selected as an ROS probe to identify the ROS produced by DBHA-NPs upon light irradiation. As shown in Fig. 5c, after DCFH-DA was incubated with the DBAH-NPs, the fluorescence intensity of the DCF becomes stronger and stronger with time from 0 to 60 min under light irradiation, indicating that the concentration of ROS increases greatly with longer incubation time. More interestingly, no fluorescence of DCF can be detected before the DBHA-NPs enter the HCT-116 cells. This is quite different from the commercial PS (porphyrin), which shows strong fluorescence both out of and inside HCT-116 cells (Fig. 5d). This phenomenon further demonstrates that DBHA-NPs can only react in tumor cells and realizes the on-site activation.
As is described above in Fig. 4c, the DBHA-NPs are mainly located in the lysosomes after entering the HCT-116 cells. Lysosomes are full of enzymes, such as hyaluronidase, which can degrade HA and increase the fluorescence intensity of the DBHA-NPs (Fig. 3d). It can be speculated that HA, the backbone of DBHA, can be degraded by hyaluronidase after the DBHA-NPs enter the lysosomes and realize fluorescence and ROS on-site activation in tumor cells. In the clinic, the on-site activation of PSs can be used in cancer diagnosis and also provides a significant strategy which doesn’t require the avoidance of light to reduce the side effects during the progress of PDT.47
Photodynamic efficiency of DBHA-NPs in vitro and in vivo
Encouraged by the above measurements, the photodynamic activities of the DBHA-NPs were further investigated against tumor cells. As shown in Fig. 6a, the DBHA-NPs show higher phototoxicity and lower dark toxicity, as well as dose-dependent survival curves, which is in good accordance with the flow cytometric assay of the DBHA-NPs (Fig. S2, ESI†). More importantly, the DBHA-NPs exhibit highly selective phototoxicity towards HCT-116 cells (CD44 overexpression) compared with A2780 cells (CD44 low expression), indicating their high targeting property towards HCT-116 cells. In contrast, HA is non-toxic towards HCT-116 cells. It can be concluded that the photodynamic activities of the DBHA-NPs originate from diiodo styryl bodipy, instead of HA. What's more, from the results of the flow cytometry assay (Fig. S3, ESI†), it can be speculated that the death mechanism of the tumor cells is mainly late-apoptotic.
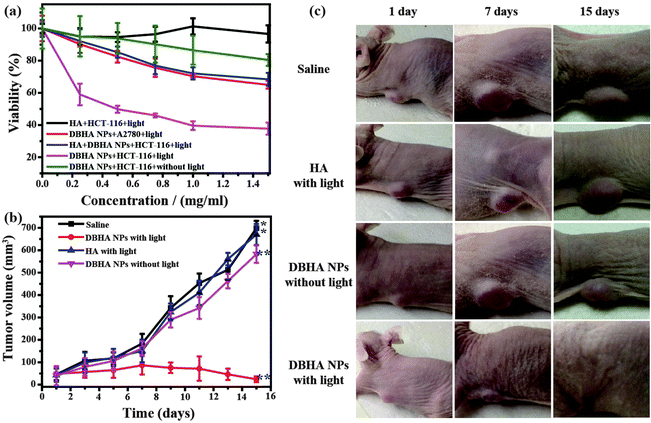 |
| Fig. 6 (a) Comparison of cell viabilities by MTT assay (λ > 600 nm). (b) The relationship between tumor volume and treatment time for the DBHA-NPs in the HCT-116 mouse model tumor cells via tail vein injection. Twenty-four mice were randomly assigned into four groups (6 mice per group), including saline with light, HA with light, DBHA-NPs without light and DBHA-NPs with light. (c) Typical photographs of tumor-bearing mice treated at different times. Note, *p < 0.05, **p < 0.01. | |
To validate the practical application of the DBHA-NPs, cancer treatments in vivo have been employed by using mice bearing HCT-116 cells. These mice were divided into four groups (6 mice in each group), which were intravenously injected with saline, HA and DBHA-NPs with or without light irradiation, followed by monitoring their tumor growth volume and body weight for fifteen days. Fig. 6b and c show the changes of tumor volume of the mice during the treatment. The results indicate that the tumor volume of mice treated with saline and HA followed by light irradiation and DBHA-NPs without light irradiation experienced a rapid growth throughout the entire treatment period. However, the tumor growth of mice treated with DBHA NPs followed by light irradiation is obviously suppressed. This suggests that the DBHA-NPs can selectively accumulate in the malignant tumor, then inhibit the tumor growth upon light irradiation through specific targeting, as well as the enhanced permeability and retention effect (“EPR” effect).48–50 Furthermore, no weight loss for the therapeutic group was observed, just the same as the controlled group (Fig. S4, ESI†), indicating that all the treatments were not severely toxic. It can be concluded that DBHA-NPs can not only serve as an efficient PS for cancer treatment, but also provide a significant strategy in the clinic to solve the problem of non-tumor site activation of PSs, further reducing the side effects for patients.
4. Conclusions
In summary, DBHA-NPs for cancer photodynamic theranostics have been successfully synthesized. Originating from the overexpression of HA receptor CD44 in tumor cells, as well as the EPR effect, the DBHA-NPs present specific targeting towards HCT-116 cells. What's more, the on-site activation of DBHA-NPs by tumors can realize exact tumor diagnosis and effectively overcome the problem of avoiding light to reduce the side effects for patients significantly. In particular, both in vitro and in vivo experiments demonstrate that DBHA-NPs can effectively suppress tumor growth, exhibiting great potential for photodynamically activatable cancer theranostics in a clinical setting.
Acknowledgements
The project was supported by 973 program (2014CB660808), Key University Science Research Project of Jiangsu Province (15KJA430006), Jiangsu Provincial Founds for Distinguished Young Scholars (BK20130046), the NNSF of China (61525402, 21275076, 61328401, 51103074), Program for New Century Excellent Talents in University (NCET-13-0853), Qing Lan Project, Synergetic Innovation Center for Organic Electronics and Information Displays, the Priority Academic Program Development of Jiangsu Higher Education Institutions (PAPD).
References
- D. E. Dolmans, D. Fukumura and R. K. Jain, Nat. Rev. Cancer, 2003, 3, 380–387 CrossRef CAS PubMed.
- D. Bechet, S. R. Mordon, F. Guillemin and M. A. Barberi-Heyob, Cancer Treat. Rev., 2014, 40, 229–241 CrossRef PubMed.
- A. Kamkaew, S. H. Lim, H. B. Lee, L. V. Kiew, L. Y. Chung and K. Burgess, Chem. Soc. Rev., 2013, 42, 77–88 RSC.
- L. Cheng, C. Wang, L. Feng, K. Yang and Z. Liu, Chem. Rev., 2014, 114, 10869–10939 CrossRef CAS PubMed.
- H. Yuan, H. Chong, B. Wang, C. Zhu, L. Liu, Q. Yang, F. Lv and S. Wang, J. Am. Chem. Soc., 2012, 134, 13184–13187 CrossRef CAS PubMed.
- C. K. Lim, J. Shin, I. C. Kwon, S. Y. Jeong and S. Kim, Bioconjugate Chem., 2012, 23, 1022–1028 CrossRef CAS PubMed.
- D. S. Kwag, N. M. Oh, Y. T. Oh, K. T. Oh, Y. S. Youn and E. S. Lee, Int. J. Pharm., 2012, 431, 204–209 CrossRef CAS PubMed.
- R. Di Corato, G. Bealle, J. Kolosnjaj-Tabi, A. Espinosa, O. Clement, A. K. Silva, C. Menager and C. Wilhelm, ACS Nano, 2015, 9, 2904–2916 CrossRef CAS PubMed.
- C. S. Shemesh, D. Moshkelani and H. Zhang, Pharm. Res., 2015, 32, 1604–1614 CrossRef CAS PubMed.
- A. Yuan, X. Tang, X. Qiu, K. Jiang, J. Wu and Y. Hu, Chem. Commun., 2015, 51, 3340–3342 RSC.
- J. Kim, O. A. Santos and J. H. Park, J. Controlled Release, 2014, 191, 98–104 CrossRef CAS PubMed.
- G. Saravanakumar, J. Lee, J. Kim and W. J. Kim, Chem. Commun., 2015, 51, 9995–9998 RSC.
- D. Ling, B. C. Bae, W. Park and K. Na, Biomaterials, 2012, 33, 5478–5486 CrossRef CAS PubMed.
- A. Kano, Y. Taniwaki, I. Nakamura, N. Shimada, K. Moriyama and A. Maruyama, J. Controlled Release, 2013, 167, 315–321 CrossRef CAS PubMed.
- J. Tu, T. Wang, W. Shi, G. Wu, X. Tian, Y. Wang, D. Ge and L. Ren, Biomaterials, 2012, 33, 7903–7914 CrossRef CAS PubMed.
- H. Benachour, A. Seve, T. Bastogne, C. Frochot, R. Vanderesse, J. Jasniewski, I. Miladi, C. Billotey, O. Tillement, F. Lux and M. Barberi-Heyob, Theranostics, 2012, 2, 889–904 CrossRef CAS PubMed.
- E. Secret, M. Maynadier, A. Gallud, A. Chaix, E. Bouffard, M. Gary-Bobo, N. Marcotte, O. Mongin, K. El Cheikh, V. Hugues, M. Auffan, C. Frochot, A. Morere, P. Maillard, M. Blanchard-Desce, M. J. Sailor, M. Garcia, J. O. Durand and F. Cunin, Adv. Mater., 2014, 26, 7643–7648 CrossRef CAS PubMed.
- Y. I. Park, H. M. Kim, J. H. Kim, K. C. Moon, B. Yoo, K. T. Lee, N. Lee, Y. Choi, W. Park, D. Ling, K. Na, W. K. Moon, S. H. Choi, H. S. Park, S. Y. Yoon, Y. D. Suh, S. H. Lee and T. Hyeon, Adv. Mater., 2012, 24, 5755–5761 CrossRef CAS PubMed.
- J. Shan, S. J. Budijono, G. Hu, N. Yao, Y. Kang, Y. Ju and R. K. Prud’homme, Adv. Funct. Mater., 2011, 21, 2488–2495 CrossRef CAS.
- C. Wang, L. Cheng, Y. Liu, X. Wang, X. Ma, Z. Deng, Y. Li and Z. Liu, Adv. Funct. Mater., 2013, 23, 3077–3086 CrossRef CAS.
- J. K. Rhee, M. Baksh, C. Nycholat, J. C. Paulson, H. Kitagishi and M. G. Finn, Biomacromolecules, 2012, 13, 2333–2338 CrossRef CAS PubMed.
- H. Kim, Y. Kim, I. H. Kim, K. Kim and Y. Choi, Theranostics, 2013, 4, 1–11 CrossRef CAS PubMed.
- W. J. Syu, H. P. Yu, C. Y. Hsu, Y. C. Rajan, Y. H. Hsu, Y. C. Chang, W. Y. Hsieh, C. H. Wang and P. S. Lai, Small, 2012, 8, 2060–2069 CrossRef CAS PubMed.
- J. Chen, T. W. Liu, P. C. Lo, B. C. Wilson and G. Zheng, Bioconjugate Chem., 2009, 20, 1836–1842 CrossRef CAS PubMed.
- T. M. Mawn, A. V. Popov, N. J. Beardsley, K. Stefflova, M. Milkevitch, G. Zheng and E. J. Delikatny, Bioconjugate Chem., 2011, 22, 2434–2443 CrossRef CAS PubMed.
- E. Clo, J. W. Snyder, N. V. Voigt, P. R. Ogilby and K. V. Gothelf, J. Am. Chem. Soc., 2006, 128, 4200–4201 CrossRef CAS PubMed.
- I. V. Nesterova, S. S. Erdem, S. Pakhomov, R. P. Hammer and S. A. Soper, J. Am. Chem. Soc., 2009, 131, 2432–2433 CrossRef CAS PubMed.
- W. Fan, W. Bu, B. Shen, Q. He, Z. Cui, Y. Liu, X. Zheng, K. Zhao and J. Shi, Adv. Mater., 2015, 27, 4155–4161 CrossRef CAS PubMed.
- C. S. Jin, L. Cui, F. Wang, J. Chen and G. Zheng, Adv. Healthcare Mater., 2014, 3, 1240–1249 CrossRef CAS PubMed.
- L. Huang, J. Zhao, S. Guo, C. Zhang and J. Ma, J. Org. Chem., 2013, 78, 5627–5637 CrossRef CAS PubMed.
- P. Yang, J. Zhao, W. Wu, X. Yu and Y. Liu, J. Org. Chem., 2012, 77, 6166–6178 CrossRef CAS PubMed.
- Y. Chen, J. Zhao, H. Guo and L. Xie, J. Org. Chem., 2012, 77, 2192–2206 CrossRef CAS PubMed.
- W. Wu, H. Guo, W. Wu, S. Ji and J. Zhao, J. Org. Chem., 2011, 76, 7056–7064 CrossRef CAS PubMed.
- L. Huang, X. Yu, W. Wu and J. Zhao, Org. Lett., 2012, 14, 2594–2597 CrossRef CAS PubMed.
- H. He, P. C. Lo, S. L. Yeung, W. P. Fong and D. K. Ng, J. Med. Chem., 2011, 54, 3097–3102 CrossRef CAS PubMed.
- M. R. Ke, S. L. Yeung, D. K. Ng, W. P. Fong and P. C. Lo, J. Med. Chem., 2013, 56, 8475–8483 CrossRef CAS PubMed.
- S. Erbas-Cakmak and E. U. Akkaya, Org. Lett., 2014, 16, 2946–2949 CrossRef CAS PubMed.
- H. J. Cho, H. Y. Yoon, H. Koo, S. H. Ko, J. S. Shim, J. H. Lee, K. Kim, I. C. Kwon and D. D. Kim, Biomaterials, 2011, 32, 7181–7190 CrossRef CAS PubMed.
- K. Y. Choi, K. H. Min, H. Y. Yoon, K. Kim, J. H. Park, I. C. Kwon, K. Choi and S. Y. Jeong, Biomaterials, 2011, 32, 1880–1889 CrossRef CAS PubMed.
- H. J. Cho, I. S. Yoon, H. Y. Yoon, H. Koo, Y. J. Jin, S. H. Ko, J. S. Shim, K. Kim, I. C. Kwon and D. D. Kim, Biomaterials, 2012, 33, 1190–1200 CrossRef CAS PubMed.
- D. S. Kwag, K. Park, K. T. Oh and E. S. Lee, Chem. Commun., 2013, 49, 282–284 RSC.
- L. Qiu, Z. Li, M. Qiao, M. Long, M. Wang, X. Zhang, C. Tian and D. Chen, Acta Biomater., 2014, 10, 2024–2035 CrossRef CAS PubMed.
- W. Miao, G. Shim, C. M. Kang, S. Lee, Y. S. Choe, H. G. Choi and Y. K. Oh, Biomaterials, 2013, 34, 9638–9647 CrossRef CAS PubMed.
- N. Kotagiri, G. P. Sudlow, W. J. Akers and S. Achilefu, Nat. Nanotechnol., 2015, 10, 370–379 CrossRef CAS PubMed.
- M. A. Sowers, J. R. McCombs, Y. Wang, J. T. Paletta, S. W. Morton, E. C. Dreaden, M. D. Boska, M. F. Ottaviani, P. T. Hammond, A. Rajca and J. A. Johnson, Nat. Commun., 2014, 5, 5460 CrossRef PubMed.
- J. Yu, C. H. Hsu, C. C. Huang and P. Y. Chang, ACS Appl. Mater. Interfaces, 2015, 7, 432–441 CAS.
- J. T. Lau, P. C. Lo, X. J. Jiang, Q. Wang and D. K. Ng, J. Med. Chem., 2014, 57, 4088–4097 CrossRef CAS PubMed.
- J. Fang, H. Nakamura and H. Maeda, Adv. Drug Delivery Rev., 2011, 63, 136–151 CrossRef CAS PubMed.
- H. Kobayashi, R. Watanabe and P. L. Choyke, Theranostics, 2013, 4, 81–89 CrossRef PubMed.
- J. W. Nichols and Y. H. Bae, J. Controlled Release, 2014, 190, 451–464 CrossRef CAS PubMed.
Footnote |
† Electronic supplementary information (ESI) available. See DOI: 10.1039/c5tb02041g |
|
This journal is © The Royal Society of Chemistry 2016 |
Click here to see how this site uses Cookies. View our privacy policy here.