DOI:
10.1039/C6AN02269C
(Paper)
Analyst, 2017,
142, 224-228
Use of β-cyclodextrin-tethered cationic polymer based fluorescence enhancement of pyrene and hybridization chain reaction for the enzyme-free amplified detection of DNA†
Received
11th October 2016
, Accepted 30th November 2016
First published on 30th November 2016
Abstract
Herein, we proposed an enzyme-free strategy for the amplified detection of DNA by combining the efficient fluorescence enhancement capability of a β-cyclodextrin-tethered cationic polymer (cationic polyβ-CD) to pyrene with the amplification capability of target DNA triggered hybridization chain reaction (HCR). Cationic polyβ-CD with positive charge was synthesized. Two hairpin probes, H1 and H2, were employed in the system and the pyrene-labelled H2 was chosen as the signal unit. The pyrene attached on the sticky end of H2 was flexible and there was strong electrostatic interaction between cationic polyβ-CD and negatively-charged H2, so pyrene could easily enter the cavity of CD that is tethered on the cationic polymer, accompanied by significant fluorescence enhancement. Once target DNA was introduced, HCR was triggered to form a rigid long dsDNA polymer with pyrene attached on it. The pyrene was hardly able to enter the cavity of cationic polyβ-CD because of steric hindrance, leading to a weak fluorescent signal. Owing to the efficient pyrene fluorescence enhancement of cationic polyβ-CD and the amplified capability of HCR, an enzyme-free sensitive detection of target DNA was achieved with a detection limit of 0.1 nM and high selectivity.
Introduction
Sensitive and selective detection of DNA is fundamental in clinical diagnosis, virus detection, biomedical studies and the development of molecular diagnostics.1 Since DNA of interest may be present in very small amounts in biological samples, many enzyme-adopted DNA amplification techniques (such as polymerase chain reaction (PCR),2 rolling circle amplification (RCA),3etc.) are widely used for achieving high sensitivity. In recent years, an enzyme-free DNA amplification technique, hybridization chain reaction (HCR), has attracted great interest;4,5 it works under isothermal conditions with a PCR-like sensitivity, avoiding the need for strict conditions to keep the activity of enzymes. In HCR, two stable species of DNA hairpin probes coexist in solution until an initiator strand is introduced. The initiator triggers a cascade of hybridization events to yield nicked double helices analogous to alternating copolymers.4,5 As an initiator-triggered reaction, it produces a comparably reduced pseudo-positive result, which means that a low background and high selectivity can be obtained.6
Nowadays, based on the initiator triggered selective polymerization of metastable hairpin DNA into a long dsDNA polymer via HCR, researchers have developed many attractive signal-amplification methods for biochemical analytical applications. For example, Willner's group7 and our group8,9 developed fluorescence methods combined with HCR for nucleic acid detection. However, some of them require complex and expensive double labelling of probes,8 and some of them need a complicated design of probes.7,9 HCR was also used for protein10–12 and ion13,14 detection. Besides, Jiang's group15 employed electrostatic nucleic acid nanoassembly through HCR in living cells for ultrasensitive mRNA imaging. Tan's group reported aptamer-tethered DNA nanotrains and nanodevices16–18 as drug carriers and cell imaging reagents. Despite the extensive research, there is still great potential use of HCR in analyses.
Because of their unique properties, analytical applications of supramolecular assemblies based on host–guest interaction have attracted considerable attraction.19 Cyclodextrin polymers (polyβ-CD) have shown efficient fluorescence emission enhancement capability during the formation of host–guest compounds with pyrene.20 The presence of multiple exterior interaction sites on polyβ-CD brings about enhanced recognition ability and forms a high affinity host–guest complex with fluorophores, associated with excellent and stable fluorescence enhancement.21–23 We have developed a DNA detection method based on the competitive host–guest interaction between electroneutral polyβ-CD (epichlorohydrin cross-linked β-cyclodextrin polymers; the synthesis was described in our previous work,21 and its zeta potential was +0.0373 mV as shown in Fig. S4†) and pyrene-labelled probes.23 Lately, we developed a series of new fluorescence methods based on the prominent fluorescence enhancement of pyrene in the presence of an electroneutral β-cyclodextrin polymer for T4 polynucleotide kinase activity,21 nucleic acid,24 microRNA,25 DNA,26 alkaline phosphatase22 and adenosine27 detection.
We have expected to develop an enzyme-free method for DNA detection that combines the prominent fluorescence enhancement capability of polyβ-CD with the amplification of target DNA triggered HCR, but the fluorescence of pyrene-labelled hairpin probes was weak in the presence of electroneutral polyβ-CD owing to the steric hindrance of hairpin probes. Lately, we synthesized a β-cyclodextrin-tethered cationic polymer (cationic polyβ-CD, i.e. the β-CD monomer was attached on poly(allylamine) through condensation; the synthesis is detailed in the ESI,† and its zeta potential was +29.2 mV as shown in Fig. S4†). Since the β-CD monomer was attached on the polymer and the electrostatic interaction between negatively-charged hairpin probes and cationic polyβ-CD was stronger than that of electroneutral polyβ-CD, pyrene could enter the cavity of cationic polyβ-CD easily, resulting in significant fluorescence enhancement. Fortunately, we found that it really has prominent fluorescence enhancement ability to pyrene labelled on the sticky end of hairpin probes. In the present work, an enzyme-free strategy for amplified detection of DNA was proposed by combining the significant fluorescence enhancement capability of cationic polyβ-CD to pyrene with the amplification capability of target DNA triggered HCR. This fluorescence amplification strategy showed highly sensitive detection of target DNA with a detection limit of 0.1 nM and high selectivity. In addition, the proposed strategy also showed great potential for applications in HCR amplified target assays.
Experimental section
Materials
Poly(allylamine) hydrochloride (PAH, Mw 65
000) and β-cyclodextrin monomer were purchased from Sigma-Aldrich Co. Polyacrylic acid (PAA), N-methyl-2-pyrrolidone (NMP) and dicyclohexylcarbodiimide (DCC, Mw 240
000) were purchased from J&K Scientific Ltd. p-Toluenesulfonyl-β-cyclodextrin (CDOTs) and monoamino-β-cyclodextrin were purchased from Chengdu Ai Keda Chemical Technology Co., Ltd. The buffer solution was Tris-HCl buffer (70 mM Tris-base, 300 mM NaCl, 10 mM MgCl2, pH 8.0). All other chemicals were of analytical grade. All stock and buffer solutions were prepared using Millipore water (18.2 MΩ cm). The oligonucleotides used in this work were ordered from TaKaRa Bio Inc. (Dalian, China). The sequences are listed in Table S1.†
Apparatus
All fluorescence measurements were carried out using an F7000 fluorometer (Hitachi, Japan) equipped with an aqueous thermostat (Amersham) accurate to 0.1 °C. Excitation and emission slits were all set for a 5.0 nm band-pass with a 700 V PMT voltage. The excitation wavelength was set at 345 nm and the emission spectra from 365 to 480 nm were collected with a 0.2 × 1 cm2 quartz cuvette containing 100 μL of solution.
Agarose electrophoresis analysis
Hairpin probes H1 and H2 were heated to 95 °C for 2 min and then allowed to cool at room temperature for at least 60 min before use. Different concentrations of target DNA were incubated with 100 nM H1 and H2 at 25 °C for 60 min. A 3% agarose gel was prepared using TAE buffer (40 mM Tris-Ac, 2.0 mM Na2EDTA, pH 8.5). SYBR Gold was used as the DNA stain and mixed with the samples. The gel was run at 60 V for 80 min at room temperature with a loading of 10 μL of sample into each lane, photographed using Gel Imaging (Tanon 2500 R, Tianneng Ltd, Shanghai, China).
Target DNA assay
In a typical DNA assay, 100 nM H1/H2 and a certain amount of target DNA were put into 100 μL Tris-HCl buffer. After incubation at 25 °C for 60 min, 0.5 mg mL−1 cationic polyβ-CD was added and the fluorescence of the mixture was measured at 25 °C.
Results and discussion
Design strategy for DNA detection
Herein, a strategy for amplified detection of DNA has been developed by combining cationic polyβ-CD with HCR. We synthesized cationic β-polyβ-CD and designed two metastable hairpin DNA probes H1 and H2 (the sequences are listed in Table S1 of the ESI,† and H2 was labelled with one pyrene on the sticky end). As illustrated in Fig. 1, in the absence of target DNA, H1 and H2 retained the hairpin state. The pyrene attached on the sticky end of H2 was flexible and there was strong electrostatic interaction between cationic polyβ-CD and negatively-charged H2 (its zeta potential was −12.85 mV), so pyrene could easily enter the cavity of CD that is tethered on the cationic polymer, resulting in significant fluorescence enhancement. However, once the target DNA was introduced, HCR was triggered to form a rigid long dsDNA polymer (its zeta potential was −11.97 mV). The pyrene attached on the rigid long dsDNA polymer was hardly able to enter the cavity of cationic polyβ-CD, leading to a weak fluorescent signal. Thus, the quantitative detection was achieved through the correlation between the concentration of target DNA and the fluorescence signal.
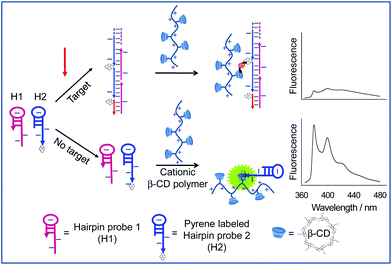 |
| Fig. 1 Schematic representation of amplified DNA detection based on β-cyclodextrin-tethered cationic polymer enhanced pyrene fluorescence and HCR. | |
The efficient pyrene fluorescence enhancement of cationic polyβ-CD
Since the pyrene fluorescence enhancement capability of polyβ-CD plays a key role in the proposed detection strategy, we first investigated the fluorescence intensity of pyrene-labelled hairpin probes H2 in the presence of cationic polyβ-CD. As a comparison, electroneutral polyβ-CD, negatively-charged polyβ-CD (the synthesis is detailed in ESI,† and its zeta potential was −50.3 mV as shown in Fig. S7†) and β-CD monomer were also investigated. As shown in Fig. 2A, the fluorescence of the system remained weak at various concentrations of β-CD monomer. It was found by our group that the fluorescence of pyrene labelled probes could be enhanced significantly by electroneutral polyβ-CD,24 while the fluorescence of the system was enhanced at most only 3 times, because the steric hindrance of the loop in H2 hinders pyrene from entering the hydrophobic cavity of the electroneutral polyβ-CD. The negatively-charged polyβ-CD also did not show significant fluorescence enhancement capability for pyrene attached on H2, because the electrostatic force prevented negatively-charged H2 from coming close to negatively-charged polyβ-CD. As for cationic polyβ-CD, the fluorescence of the system could increase by more than 10 times (Fig. 2B), because pyrene could easily act with polyβ-CD owing to the strong electrostatic interaction between cationic polyβ-CD and negatively-charged H2. Thus cationic polyβ-CD was chosen for further studies. The efficient fluorescence enhancement capability of cationic polyβ-CD was expected to improve the sensitivity of the sensing strategy.
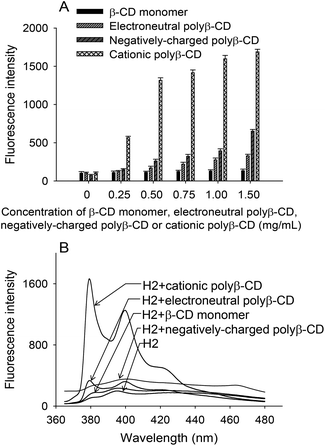 |
| Fig. 2 (A) Fluorescence intensity of 100 nM pyrene-labelled H2 and various concentrations of β-CD monomer, electroneutral polyβ-CD, negatively-charged polyβ-CD or cationic polyβ-CD. Error bars indicate the standard deviations for three experiments. (B) Fluorescence spectra of 100 nM pyrene-labelled H2 and β-CD monomer, electroneutral polyβ-CD, negatively-charged polyβ-CD or cationic polyβ-CD. The concentrations of β-CD monomer, electroneutral polyβ-CD, and cationic polyβ-CD were 1.5 mg mL−1, and the concentration of electroneutral polyβ-CD was 0.5 mg mL−1. | |
The feasibility of the strategy
The feasibility of our strategy was then investigated and shown in Fig. 3A. In the absence of target DNA, the fluorescence of the system was enhanced greatly. When the target DNA was introduced, it could trigger the HCR process and the formation of a rigid long dsDNA polymer, with pyrene attached on it. The pyrene was hardly able to enter the cavity of cationic polyβ-CD because of steric hindrance, so the fluorescence of the system was weak. In comparison, we also investigated the fluorescence spectra of the system in the presence of electroneutral polyβ-CD and negatively-charged polyβ-CD (Fig. 3B and C); the fluorescence intensity of pyrene in the presence or absence of target DNA has slight distinction because of the limited fluorescence enhancement ability of electroneutral polyβ-CD and negatively-charged polyβ-CD. The results proved that electrostatic interactions also played an important role for it made DNA (dsDNA or H2) remain close to cationic polyβ-CD, so host–guest interaction between cationic polyβ-CD and pyrene attached on DNA became possible. In the case of dsDNA, the steric hindrance of the rigid dsDNA polymer prevents host–guest interaction, while in the case of hairpin probe H2, pyrene attached on the flexible sticky end of H2 could easily enter the cavity, accompanied by fluorescence enhancement. The target DNA-triggered HCR was also examined by gel electrophoresis. As shown in Fig. 3D, in the absence of target DNA, there were only a low molecular weight band, indicating that no HCR occurred. Once the target DNA was introduced, smears of high molecular weight structures were observed. This indicated that target DNA triggered the HCR process between H1 and H2 to form the long dsDNA polymer and the weight of the dsDNA polymer was inversely related to the concentration of the target DNA given the fixed hairpin probe supply.
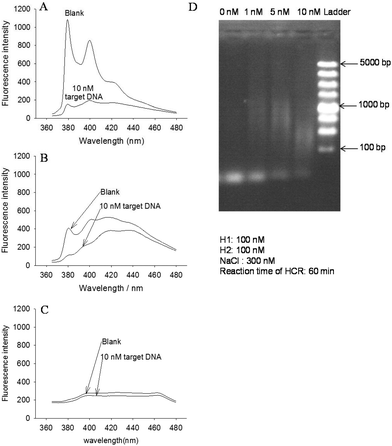 |
| Fig. 3 (A) Fluorescence spectra of the system in the presence of 0.5 mg mL−1 cationic polyβ-CD. (B) Fluorescence spectra of the system in the presence of 1.5 mg mL−1 electroneutral polyβ-CD. (C) Fluorescence spectra of the system in the presence of 1.5 mg mL−1 negatively-charged polyβ-CD. (D) Agarose gel (3%) electrophoresis image. The concentration of target DNA was 0, 1, 5 and 10 nM respectively. The concentrations of H1 and H2 were 100 nM respectively. The concentration of NaCl was 300 nM. The reaction time of HCR was 60 min. | |
Analytical performance of DNA detection
To characterize the detection range of target DNA, the fluorescence emission spectra of the system containing various amounts of target DNA were measured under optimized conditions (the details of the optimization are given in the ESI†). As shown in Fig. 4A, the fluorescence intensity of the system decreased with an increase of target DNA from 0 to 20 nM. A linear dependence of (F0 − F)/F0 on target DNA in the range of 0.1–2.0 nM was found (Fig. 4B, insert). The detection limit was 0.1 nM (S/N = 3) and R2 = 0.9918.
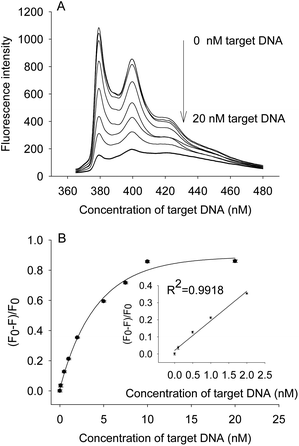 |
| Fig. 4 (A) Fluorescence spectra of the detection system at various concentrations of target DNA (from top to bottom): 0, 0.1, 0.5, 1.0, 2.0, 5.0, 7.0, 10.0, and 20.0 nM. (B) Plot of target DNA vs. (F0 − F)/F0 (at 380 nm). The inset is the calibration curve for the concentration of target DNA from 0 to 2.0 nM. The excitation wavelength was set at 345 nm. The concentrations of H1 and H2 were 100 nM. The concentrations of cationic polyβ-CD and NaCl were 0.5 mg mL−1 and 300 nM respectively. The reaction time of HCR was 60 min. Error bars indicate the standard deviations for three experiments. | |
As an analytical method, high specificity was a matter of necessity. The specificity was tested by measuring and comparing the response of target DNA to single-base mismatched DNA (Mismatched DNA), single-base deleted DNA (Deleted DNA) and single-base inserted DNA (Inserted DNA) respectively. As shown in Fig. 5, the value of (F0 − F)/F0 was above 0.8 in the presence of target DNA, while in the presence of control DNA, (F0 − F)/F0 was low. Because the control DNA sequences were hardly able to initialize the HCR, only the perfectly matched DNA triggered the HCR process efficiently, so the high specificity was achieved.
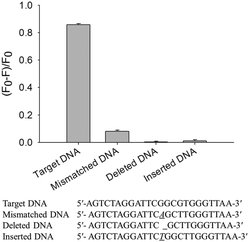 |
| Fig. 5 Specificity investigation for target DNA against Mismatched DNA, Deleted DNA and Inserted DNA. The concentrations of various DNA were 10 nM. The excitation wavelength was set at 345 nm. The concentrations of H1 and H2 were 100 nM, respectively. The concentrations of cationic polyβ-CD and NaCl were 0.5 mg mL−1 and 300 nM respectively. The reaction time of HCR was 60 min. Error bars indicate the standard deviations for three experiments. | |
Conclusions
In conclusion, we have synthesized cationic polyβ-CD and successfully developed a sensitive and selective DNA detection method based on the prominent fluorescence enhancement capability of cationic polyβ-CD to pyrene labelled hairpin probes and HCR. This strategy offered several advantages: (1) owing to the prominent fluorescence enhancement capability of cationic polyβ-CD, sensitive detection of target DNA without the aid of enzyme was achieved with a detection limit of 0.1 nM; (2) owing to the target DNA triggered HCR, it achieved high specificity; (3) it avoided expensive double-labeling and the labeling of the quencher, so we did not bother about the distance between the quencher and the fluorophore. It provides a new opportunity for DNA assays.
Acknowledgements
This work was supported by the National Natural Science Foundation of China (21190044 and 21675047) and the National Basic Research Program of China (2011CB911002).
Notes and references
- H. Zhang, F. Li, B. Dever, X.-F. Li and X. C. Le, Chem. Rev., 2013, 113, 2812–2841 CrossRef CAS PubMed.
- R. Saiki, D. Gelfand, S. Stoffel, S. Scharf, R. Higuchi, G. Horn, K. Mullis and H. Erlich, Science, 1988, 239, 487–491 CAS.
- F. Barany, Proc. Natl. Acad. Sci. U. S. A., 1991, 88, 189–193 CrossRef CAS.
- R. M. Dirks and N. A. Pierce, Proc. Natl. Acad. Sci. U. S. A., 2004, 101, 15275–15278 CrossRef CAS PubMed.
- Y. Zhao, F. Chen, Q. Li, L. Wang and C. Fan, Chem. Rev., 2015, 115, 12491–12545 CrossRef CAS PubMed.
- J. Huang, X. Yang, X. He, K. Wang, Y. He and K. Quan, Chem. Commun., 2013, 49, 9827–9829 RSC.
- F. Wang, J. Elbaz, R. Orbach, N. Magen and I. Willner, J. Am. Chem. Soc., 2011, 133, 17149–17151 CrossRef CAS PubMed.
- J. Huang, Y. Wu, Y. Chen, Z. Zhu, X. Yang, C. J. Yang, K. Wang and W. Tan, Angew. Chem., Int. Ed., 2011, 50, 401–404 CrossRef CAS PubMed.
- J. Huang, H. Wang, X. Yang, K. Quan, Y. Yang, L. Ying, N. Xie, M. Ou and K. Wang, Chem. Sci., 2016, 7, 3829–3835 RSC.
- B. Zhang, B. Liu, D. Tang, R. Niessner, G. Chen and D. Knopp, Anal. Chem., 2012, 84, 5392–5399 CrossRef CAS PubMed.
- J. Choi, K. Routenberg Love, Y. Gong, T. M. Gierahn and J. C. Love, Anal. Chem., 2011, 83, 6890–6895 CrossRef CAS PubMed.
- J. Zhou, M. Xu, D. Tang, Z. Gao, J. Tang and G. Chen, Chem. Commun., 2012, 48, 12207–12209 RSC.
- J. Huang, X. Gao, J. Jia, J.-K. Kim and Z. Li, Anal. Chem., 2014, 86, 3209–3215 CrossRef CAS PubMed.
- J. Zhuang, L. Fu, M. Xu, Q. Zhou, G. Chen and D. Tang, Biosens. Bioelectron., 2013, 45, 52–57 CrossRef CAS PubMed.
- Z. Wu, G. Q. Liu, X. L. Yang and J. H. Jiang, J. Am. Chem. Soc., 2015, 137, 6829–6836 CrossRef CAS PubMed.
- G. Z. Zhu, J. Zheng, E. Q. Song, M. Donovan, K. J. Zhang, C. Liu and W. H. Tan, Proc. Natl. Acad. Sci. U. S. A., 2013, 110, 7998–8003 CrossRef CAS PubMed.
- G. Z. Zhu, S. F. Zhang, E. Q. Song, J. Zheng, R. Hu, X. H. Fang and W. H. Tan, Angew. Chem., Int. Ed., 2013, 52, 5490–5496 CrossRef CAS PubMed.
- S. Cansiz, L. Zhang, C. Wu, Y. Wu, I. T. Teng, W. Hou, Y. Wang, S. Wan, R. Cai, C. Jin, Q. Liu and W. Tan, Chem. – Asian J., 2015, 10, 2084–2094 CrossRef CAS PubMed.
- L. Szente and J. Szemán, Anal. Chem., 2013, 85, 8024–8030 CrossRef CAS PubMed.
- M. Hollas, M.-A. Chung and J. Adams, J. Phys. Chem. B, 1998, 102, 2947–2953 CrossRef CAS.
- C. Song, X. Yang, K. Wang, Q. Wang, J. Liu, J. Huang, L. He, P. Liu, Z. Qing and W. Liu, Chem. Commun., 2015, 51, 1815–1818 RSC.
- C. Song, X. Yang, K. Wang, Q. Wang, J. Liu, J. Huang, M. Zhou and X. Guo, Spectrochim. Acta, Part A, 2016, 156, 131–137 CrossRef CAS PubMed.
- P. Liu, S. Sun, X. Guo, X. Yang, J. Huang, K. Wang, Q. Wang, J. Liu and L. He, Anal. Chem., 2015, 87, 2665–2671 CrossRef CAS PubMed.
- X. Guo, P. Liu, X. Yang, K. Wang, Q. Wang, Q. Guo, J. Huang, J. Liu, C. Song and W. Li, Analyst, 2015, 140, 2016–2022 RSC.
- X. Guo, X. Yang, P. Liu, K. Wang, Q. Wang, Q. Guo, J. Huang, W. Li, F. Xu and C. Song, Analyst, 2015, 140, 4291–4297 RSC.
- H. Huang, X. Yang, K. Wang, Q. Wang, Q. Guo, J. Huang, J. Liu, X. Guo, W. Li and L. He, Talanta, 2015, 144, 529–534 CrossRef CAS PubMed.
- H. Huang, X. Yang, K. Wang, Q. Wang, Q. Guo, J. Huang, J. Liu and C. Song, Analyst, 2016, 141, 2502–2507 RSC.
Footnote |
† Electronic supplementary information (ESI) available: More experimental details and additional figures as noted in the text. See DOI: 10.1039/c6an02269c |
|
This journal is © The Royal Society of Chemistry 2017 |
Click here to see how this site uses Cookies. View our privacy policy here.