DOI:
10.1039/C6AY02477G
(Communication)
Anal. Methods, 2017,
9, 23-27
Quantitative determination of calcium ions by means of enhanced fluorescence of silver nanocluster complex†
Received
3rd September 2016
, Accepted 8th November 2016
First published on 17th November 2016
Abstract
A simple and green method for the synthesis of fluorescent silver nanocluster–nanoparticle complexes (AgNCs–AgNPs) is presented. In this method, Ag+ was reduced to form AgNCs–AgNPs using hydrogen as the reductant and sodium polyacrylate as a soft template at room temperature. The AgNCs–AgNPs possess strong fluorescence, and the fluorescence property has high sensitivity and excellent selectivity to Ca2+. An approach based on the enhancement of fluorescence was firstly applied for the quantitative determination of Ca2+. The approach displays a wide linear response, excellent selectivity and high sensitivity, and provides a potential candidate for the quantitative determination of Ca2+ in biological systems.
Various ions play important roles in the bio-system, for example, in signaling. An important ion related to cellular signaling is Ca2+, which plays an indispensable role in regulating several biological processes. These roles include excitability, neurotransmitter release, gene transcription, cell proliferation, synaptic plasticity, and hormone secretion.1–3 Moreover, some anomalous variations of several biological processes can be estimated by monitoring the Ca2+ level in serum, which has to be strictly maintained in a narrow range in the bio-system. Therefore, an abnormal serum Ca2+ level might be detected and diagnosed early by detecting the Ca2+ concentration.4,5 For these reasons, numerous probes (organic dyes,6,7 protein,8,9 magnetic nanoparticles,10 gold nanoparticles (AuNPs)11) for measuring the concentration of Ca2+ have been developed in recent decades. Particularly, fluorescence probes are significantly useful in the quantitative determination of Ca2+ in the biomedical field because of their high sensitivity and precision. A large variety of ion-sensitive fluorophores can be obtained by changing their fluorescence intensity in the presence of the relevant ions.12–14 With such fluorophores, intracellular ion concentrations could, in principle, be determined, which would be an interesting tool for cell biology.15–19 Most Ca2+ sensitive fluorophores are based on organic molecules14 or fluorescent nanoparticles (carbon dots18 and quantum dots19). However, the organic dyes and fluorescent nanoparticles may not only be toxic to biological systems, but also cause fluorescence quenching. Moreover, the use of particle-based intracellular sensors for Ca2+ detection is not straight forward. Important associated problems involve crosstalk of the fluorescence read-out with pH and spectral overlap of the emission spectra of different fluorophores. Alternatively, colloidal particles with a fluorescence read-out are commonly used as sensors for the quantitative determination of ions. The ion-sensitive probes would allow the spatiotemporal observation of such signaling dynamics. Colloidal particles of silver nanoclusters (Ag NCs), as a promising chemical sensor, have been used as a probe to detect bio-macromolecules (e.g., DNA, RNA and proteins),20,21 and heavy metal ions (e.g., Hg2+, Cu2+, Cr3+, Al3+).22–24 In the past decades, Ag NCs have been synthesized by various methods including chemical reduction,25 photo-generation,26–28 ultrasonic reduction29 and ligand etching.30,31 Nevertheless, the Ag NCs synthesized by these methods suffer from a highly complex synthetic process and high cost, which has limited the quantitative determination of Ca2+ in the biomedical field. Therefore, designing an easier and green way for the generation of fluorescent Ag NCs with good biocompatibility is still a worthwhile and challenging task.
In this communication, for the first time, a simple method to synthesize fluorescent AgNCs–AgNPs with excellent fluorescence and good stability was explored using hydrogen (H2)32 as a reducing agent and sodium polyacrylate (PAA) as a soft template at room temperature (Scheme 1). The morphology and size of the AgNCs–AgNPs were determined and the optical properties were demonstrated. The interaction between the AgNCs–AgNPs and Ca2+ was studied. Based on the relation of AgNCs and Ca2+, a method for the quantitative determination of Ca2+ concentrations was established and the possible impact factors were analysed.
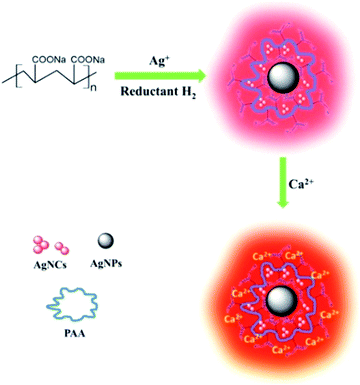 |
| Scheme 1 Illustration of the synthesis of AgNCs and the detection of Ca2+ with the prepared AgNCs. | |
A green and simple method was established to synthesize fluorescent AgNCs–AgNPs. In the synthetic process, Ag+ was reduced by H2 using PAA as a soft template at room temperature. The complexes were synthesized by dissolving AgNO3 in an aqueous solution of PAA to form a PAA–Ag solution. Then, H2 was passed through the PAA–Ag solution at room temperature to synthesize fluorescent AgNCs–AgNPs. The colorless PAA–Ag solution gradually changed along with the addition of H2 by turning pink at 20 min and dark purple at 60 min. H2 as the reductant not only enables the nanoparticle to be synthesized with a uniform size, but also is used to avoid residual amounts of the reductant. The detailed synthetic procedure can be found in ESI.† The effect of various factors (Ag+ concentration, ratio of [COO−]/[Ag+] and H2 pressure) on the fluorescence of the complexes was studied, and the results are shown in Fig. S1–3 of the ESI.† The optimal conditions to synthesize fluorescent AgNCs–AgNPs were obtained (ESI†).
The UV-visible absorption, excitation and emission spectra of AgNCs–AgNPs are presented in Fig. 1. The maximum UV absorption wavelength of the complexes is about 500 nm, which is totally different from that of AgNPs (400 nm), but it is the same as a majority of AgNCs.27,29,33–36 With the increase in reaction time, the UV absorbance intensity was enhanced and the width at half maximum intensity was increased, as shown in Fig. 1a. Fluorescence emission spectra of the complexes at an excitation wavelength of 520 nm are shown in Fig. 1b. In the first 20 minutes, the fluorescence intensity increases with the reaction time (Fig. 1c). It indicates that the fluorescent AgNCs were formed gradually. However, after about a 20 min reaction, a red shift of the maximum emission wavelength of the complexes with was observed for with increase in reaction time (Fig. 1b). This may be ascribed to the formation of AgNCs–AgNPs as shown in Fig. 1d, which depends on the ratio of AgNCs to AgNPs in the complexes. The emission intensity of the complexes decreased with an increase in reaction time in the range of 20 min to 60 min (Fig. 1c). These results might be generated from the distribution of the number of Ag atoms in the AgNCs and the strong coupling between the emission of AgNCs and the surface plasma resonance of AgNPs.37–40 The SEM images of AgNCs–AgNPs prepared at 20 min and 60 min are shown in Fig. S4 ESI.† The particle size prepared at 20 min was smaller than that at 60 min. The average diameter of the particles after a 20 min reaction was about 3.0 nm, as shown in the TEM images (Fig. 1d and e). The abovementioned results indicated that the fluorescent nanoparticles could be AgNCs–AgNPs.
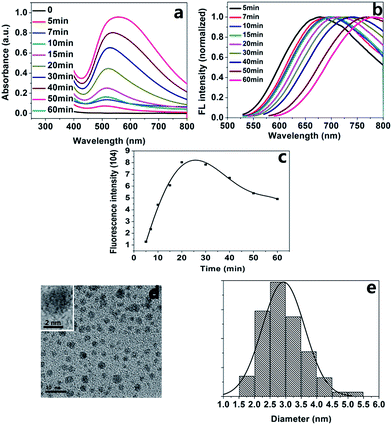 |
| Fig. 1 (a) Absorbance spectra of the AgNCs–AgNPs complexes at various reaction times (0–60 min); (b) the fluorescence spectra (normalized) of the AgNCs–AgNPs at various reaction times at an excitation wavelength of 520 nm in the first 20 minutes; (c) the relationship of the fluorescence intensity of the complexes to reaction time; (d) TEM image of the complexes. The scale bar is 10 nm and 2 nm for (d) and the inset, respectively; (e) the particle size distribution histogram. | |
AgNCs–AgNPs were synthesized at optimal conditions using the abovementioned method. The UV-visible absorption and fluorescence spectra of the AgNCs–AgNPs are shown in Fig. 2a. The maximum absorption (500 nm) of the complexes is the characteristic absorption band of the AgNCs. It indicates that the optical properties come from the AgNCs. The emission spectra (normalized) of the complexes at various excitation wavelengths are shown in Fig. 2b. The complexes have an intense fluorescence. With an increase in the excitation wavelength, the intensity increased in the range of 480–520 nm; however, it decreased beyond 520 nm (Fig. 2c). The intense fluorescence is most likely due to their lower density of electronic states.41 A red shift of the emission wavelength with an increase of the excitation wavelength from 480 nm to 560 nm was observed. It might be generated from the strong coupling between the emission of AgNCs and the surface plasma resonance of AgNPs.
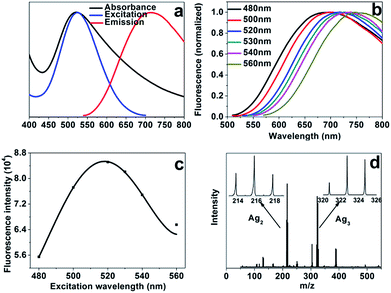 |
| Fig. 2 (a) The absorbance, excitation and fluorescence spectra of AgNCs–AgNPs. Black line, blue line and red line represent absorbance, excitation and emission, respectively; (b) the emission spectra (normalized) of the AgNCs–AgNPs at various excitation wavelengths; (c) the relationship of fluorescence intensity of AgNCs–AgNPs to the excitation wavelength; (d) the number of Ag atoms in AgNCs–AgNPs. | |
Matrix-assisted laser desorption ionisation time-off flight mass spectrometry (MALDI-TOF-MS)42 was used to probe AgNCs and analyse the distribution of the number of Ag atoms in the complexes (Fig. 2d). The results suggested the presence of a distribution of AgNCs with varying number of Ag atoms, and the complexes comprised mainly of Ag2 and Ag3 atoms clusters.42–44 The luminescence lifetime of AgNCs–AgNPs was measured by the method of time-correlated single-photon counting and it was 1.6 ± 0.03 ns. Such a short lifetime is consistent with a previous report for the water-soluble AgNCs26,45 and it suggests that the fluorescence of the complexes mainly comes from AgNCs. The photostability of the complexes was studied, and the results demonstrated that the fluorescence intensity of the complexes remained unchanged essentially after several weeks of storage in the dark (under 4 °C).
It is noteworthy that the interaction between the prepared AgNCs–AgNPs and Ca2+ is prominent at certain Ca2+ concentrations. The relationship between AgNCs–AgNPs and Ca2+ concentration was studied using fluorescence and UV spectroscopy, as shown in Fig. S5 and S6 of ESI,† respectively. The fluorescence spectra of the complexes in solutions of various Ca2+ concentrations are shown in Fig. 3a. The emission intensity of the AgNCs–AgNPs system increased with the Ca2+ concentration increasing in the region below 3.85 mM and the intensity was about three times more than that of the AgNCs–AgNPs without Ca2+. The zeta potentials of the composite system were obtained by dynamic light scattering measurements, and increased with an increase in the Ca2+ concentration, as shown in Fig. S4.† The relationship between the fluorescence intensity of the composite system at various Ca2+ concentrations and the zeta potentials is shown in Fig. 3b. This is most likely due to Ca2+ ions connecting the carboxylate groups on the surface of the complexes, which may optimize the surface state of AgNCs–AgNPs and can impact the process of LMMCT (ligand-to-metal-metal charge transfer) in AgNCs-AgNPs complexes that generated the fluorescence.45
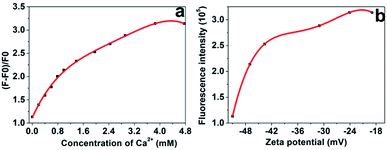 |
| Fig. 3 (a) The fluorescence emission intensity of the composite system with various Ca2+ concentrations at an excitation wavelength of 520 nm; (b) relationship between fluorescence intensity of the composite system with various concentrations of Ca2+ and the zeta potential. | |
There is a low Ca2+ concentration (0.94–1.33 mM) in human serum and the variation of the Ca2+ concentration in human serum may indicate some biological processes that are related to some diseases.4,5 In order to determine the Ca2+ concentration using the AgNCs–AgNPs as a fluorescence probe, the relationship of AgNCs–AgNPs to Ca2+ was studied. A good linear relationship between the intensity ratio ((F − F0)/F0; where F and F0 are the fluorescence intensity of fluorescence probe in the presence and absence of Ca2+) of AgNCs–AgNPs and Ca2+ concentrations was found. The correlation coefficient R2 of the calibration curve is 0.9890 in the Ca2+ concentration range from 0.20 mM to 1.19 mM (Fig. 4a).
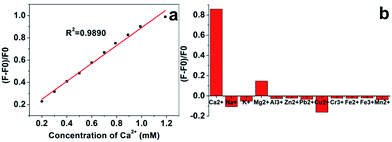 |
| Fig. 4 (a) Linear relationship of intensity ratio ((F − F0)/F0) of the fluorescence probe vs. Ca2+ concentrations; (b) the selectivity of the fluorescence probe toward Ca2+ over other metal ions in water. The concentration of the metal ions was 1.0 mM, 0.14 M, 4.0 mM, 1.0 mM, 0.07 mM, 15 μM, 0.48 μM, 20 μM, 14 μM, 20 μM, 4 μM and 0.1 mM for Ca2+, Na+, K+, Mg2+, Al3+, Zn2+, Pb2+, Cu2+, Cr3+, Fe2+, Fe3+ and Mn2+, respectively. | |
Artificial blood was used as a blood substitute to investigate the selectivity of this fluorescence probe for the determination of Ca2+ concentrations. A series of interferential experiments was carried out to evaluate Ca2+ selective fluorescence responses in the presence of various cations as competitors. Most of the important metal cations in human serum, such as Na+, K+, Mg2+, Al3+, Zn2+, Pb2+, Cu2+, Cr3+, Fe2+, Fe3+ and Mn2+ were tested, in which 1.0 mM of Ca2+ in common artificial blood was used to divide indicator standards, and concentrations of other cations were fixed at common levels in artificial blood, as shown in Fig. 4. The selectivity of the fluorescence probe on Ca2+ was compared and is displayed in Fig. 4b. Compared with the intensity change of the complex with Ca2+, intensity changes with other ions were really small as shown in Fig. 4b. The result of the selectivity test showed that the AgNCs–AgNPs had a selectivity toward Ca2+ in a certain concentration range. This means that the AgNCs–AgNPs probe can be used to quantitatively determine Ca2+ concentrations.
Conclusions
A convenient method to synthesize water-soluble fluorescent AgNCs–AgNPs was presented. In the synthetic process, Ag+ was reduced by hydrogen (H2) using sodium polyacrylate as a soft template at room temperature. The AgNCs–AgNPs possessed a strong fluorescence intensity, good light stability, and these features could greatly extend the scope of their application. The excellent fluorescence property has good sensitivity to Ca2+. The Ca2+ sensing system was constructed for the quantitative determination of Ca2+ concentrations. The method not only displays a wide linear response, low detection limit, excellent selectivity and high sensitivity, but also has the advantages of low cost and facile fabrication. The method provides a potential candidate for the quantitative determination of calcium ions in biosystems.
Acknowledgements
This research was supported by the National Natural Science Foundation of China (21473055, and 21273073), the National High-Tech R&D (863) Program of China (2011AA06A107), and the Fundamental Research Funds for the Central Universities (No. WJ1516001).
Notes and references
- A. Marty, Trends Neurosci., 1989, 12, 420–424 CrossRef CAS PubMed.
- R. C. Malenka, J. A. Kauer, D. J. Perkel and R. A. Nicoll, Trends Neurosci., 1989, 12, 444–450 CrossRef CAS PubMed.
- S. Orrenius, B. Zhivotovsky and P. Nicotera, Nat. Rev. Mol. Cell Biol., 2003, 4, 552–565 CrossRef CAS PubMed.
- G. E. Breitwieser, Int. J. Biochem. Cell Biol., 2008, 40, 1467–1480 CrossRef CAS PubMed.
- N. Solovyova and A. Verkhratsky, J. Neurosci. Methods, 2002, 122, 1–12 CrossRef CAS PubMed.
- V. K. Johns, P. K. Patel, S. Hassett, P. Calvo-Marzal, Y. Qin and K. Y. Chumbimuni-Torres, Anal. Chem., 2014, 86, 6184–6187 CrossRef CAS PubMed.
- X. Xie, J. Zhai, G. A. Crespo and E. Bakker, Anal. Chem., 2014, 86, 8770–8775 CrossRef CAS PubMed.
- H. Yuan, C. Xing, H. An, R. Niu, R. Li, W. Yan and Y. Zhan, ACS Appl. Mater. Interfaces, 2014, 6, 14790–14794 CAS.
- Y. Wang, L. Tang, W. Liu, Y. Zhao, B. G. Oscar, R. E. Campbell and C. Fang, J. Phys. Chem. B, 2015, 119, 2204–2218 CrossRef CAS PubMed.
- S. Taktak, R. Weissleder and L. Josephson, Langmuir, 2008, 24, 7596–7598 CrossRef CAS PubMed.
- S. Kim, J. Kim, N. H. Lee, H. H. Jang and M. S. Han, Chem. Commun., 2011, 47, 10299–10301 RSC.
- R. D. Carpenter and A. S. Verkman, Org. Lett., 2010, 12, 1160–1163 CrossRef CAS PubMed.
- M. J. Ruedas-Rama, A. Orte, E. A. Hall, J. M. Alvarez-Pez and E. M. Talavera, Analyst, 2012, 137, 1500–1508 RSC.
- W. Jiang, Q. Fu, H. Fan and W. Wang, Chem. Commun., 2008, 259–261, 10.1039/b712377a.
- P. R. Gil, M. Nazarenus, S. Ashraf and W. J. Parak, Small, 2012, 8, 943–948 CrossRef PubMed.
- T. Mistri, M. Dolai, D. Chakraborty, A. R. Khuda-Bukhsh, K. K. Das and M. Ali, Org. Biomol. Chem., 2012, 10, 2380–2384 CAS.
- X.-A. Zhang, D. Hayes, S. J. Smith, S. Friedle and S. J. Lippard, J. Am. Chem. Soc., 2008, 130, 15788–15789 CrossRef CAS PubMed.
- A. S. Krishna, C. Radhakumary and K. Sreenivasan, Analyst, 2013, 138, 7107–7111 RSC.
- V. Yadav, R. A. Pavlick, S. M. Meckler and A. Sen, Chem. Mater., 2014, 26, 4647–4652 CrossRef CAS.
- Y. Xiao, Z. Wu, K. Y. Wong and Z. Liu, Chem. Commun., 2014, 50, 4849–4852 RSC.
- J. Li, J. You, Y. Zhuang, C. Han, J. Hu, A. Wang, K. Xu and J. J. Zhu, Chem. Commun., 2014, 50, 7107–7110 RSC.
- Z. Yuan, N. Cai, Y. Du, Y. He and E. S. Yeung, Anal. Chem., 2014, 86, 419–426 CrossRef CAS PubMed.
- T. Y. Zhou, L. P. Lin, M. C. Rong, Y. Q. Jiang and X. Chen, Anal. Chem., 2013, 85, 9839–9844 CrossRef CAS PubMed.
- S. Ghosh, U. Anand and S. Mukherjee, Anal. Chem., 2014, 86, 3188–3194 CrossRef CAS PubMed.
- K. Zheng, X. Yuan, K. Kuah, Z. Luo, Q. Yao, Q. Zhang and J. Xie, Chem. Commun., 2015, 51, 15165–15168 RSC.
- L. Shang and S. Dong, Chem. Commun., 2008, 1088–1090 RSC.
- B. D. Martin, J. Fontana, Z. Wang, J. Louis-Jean and S. A. Trammell, Chem. Commun., 2012, 48, 10657–10659 RSC.
- L. Maretti, P. S. Bilone, Y. Liu and J. C. Scaiano, J. Am. Chem. Soc., 2009, 131, 13972–13980 CrossRef CAS PubMed.
- H. Xu and K. S. Suslick, ACS Nano, 2010, 4, 3209–3214 CrossRef CAS PubMed.
- T. Udaya Bhaskara Rao and T. Pradeep, Angew. Chem., 2010, 49, 3925–3929 CrossRef CAS PubMed.
- K. Siriwardana, N. Suwandaratne, G. S. Perera, W. E. Collier, F. Perez and D. Zhang, J. Phys. Chem. C, 2015, 119, 20975–20984 CAS.
- K. D. Bhatte, K. M. Deshmukh, Y. P. Patil, D. N. Sawant, S.-I. Fujita, M. Arai and B. M. Bhanage, Particuology, 2012, 10, 140–143 CrossRef CAS.
- L. Shang and S. Dong, Chem. Commun., 2008, 1088–1090, 10.1039/b717728c.
- H.-T. Hsieh, W.-K. Chin and C.-S. Tan, Langmuir, 2010, 26, 10031–10035 CrossRef CAS PubMed.
- L. Lu and X. An, J. Supercrit. Fluids, 2015, 99, 29–37 CrossRef CAS.
- X. Peng, J. Wickham and A. P. Alivisatos, J. Am. Chem. Soc., 1998, 120, 5343–5344 CrossRef CAS.
- J. Bellessa, C. Bonnand, J. C. Plenet and J. Mugnier, Phys. Rev. Lett., 2004, 93, 036404 CrossRef CAS PubMed.
- A. Salomon, R. J. Gordon, Y. Prior, T. Seideman and M. Sukharev, Phys. Rev. Lett., 2012, 109, 073002 CrossRef PubMed.
- D. E. Gomez, K. C. Vernon, P. Mulvaney and T. J. Davis, Nano Lett., 2010, 10, 274–278 CrossRef CAS PubMed.
- A. Berrier, R. Cools, C. Arnold, P. Offermans, M. Crego-Calama, S. H. Brongersma and J. Gómez-Rivas, ACS Nano, 2011, 8, 6226–6232 CrossRef PubMed.
- J. Zheng, C. Zhang and R. M. Dickson, Phys. Rev. Lett., 2004, 93, 077402 CrossRef PubMed.
- M. Ganguly, A. Pal, Y. Negishi and T. Pal, Langmuir, 2013, 29, 2033–2043 CrossRef CAS PubMed.
- Y. Bao, C. Zhong, D. M. Vu, J. P. Temirov, R. B. Dyer and J. S. Martinez, J. Phys. Chem. C, 2007, 111, 12194–12198 CAS.
- Z. Shen, H. Duan and H. Frey, Adv. Mater., 2007, 19, 349–352 CrossRef CAS.
- Y. Chen, T. Yang, H. Pan, Y. Yuan, L. Chen, M. Liu, K. Zhang, S. Zhang, P. Wu and J. Xu, J. Am. Chem. Soc., 2014, 136, 1686–1689 CrossRef CAS PubMed.
Footnote |
† Electronic supplementary information (ESI) available. See DOI: 10.1039/c6ay02477g |
|
This journal is © The Royal Society of Chemistry 2017 |
Click here to see how this site uses Cookies. View our privacy policy here.