DOI:
10.1039/C6BM00706F
(Communication)
Biomater. Sci., 2017,
5, 50-56
Gadolinium hybrid iron oxide nanocomposites for dual T1- and T2-weighted MR imaging of cell labeling
Received
4th October 2016
, Accepted 30th October 2016
First published on 14th November 2016
Abstract
Tracking of cells in biological systems is critically important for monitoring disease treatment, such as in stem cell therapy. This report prepared new types of biocompatible gadolinium hybrid iron oxide (GdIO) nanocomposites, which demonstrated high sensitivity for dual T1- and T2-weighted magnetic resonance imaging (MRI). The GdIO nanocomposites could efficiently label mesenchymal stem cells (MSCs) by incubation for 24 h at a safe dose, as they did not affect the cell viability, proliferation or differentiation capacity. There was high contrast enhancement in the GdIO-labeled stem cells for dual T1- and T2-weighted MR imaging. In addition, the GdIO nanocomposites were injected into adult mouse lateral ventricles, where cells could be labeled to monitor their biological behaviors by MRI. These GdIO nanocomposites with dual-imaging functions are a good platform for cell labeling and other diagnostic applications.
Introduction
Stem cells such as mesenchymal stem cells (MSCs) are multipotent stromal cells, which can differentiate into a variety of cell types, including osteoblasts (e.g., bone cells), chondrocytes (e.g., cartilage cells), and adipocytes (e.g., fat cells).1,2 In the past decades, MSCs have played an important role in the treatment of damaged tissues by directly differentiating into many different resident types of cells and/or by secreting trophic factors to enhance tissue repair,3–5 demonstrating achievements in treating many diseases. However, the wide clinical applications of stem cells are still limited because the cellular behaviors are not adequately understood. Thus, there is a clinical demand to apply a suitable method to noninvasively investigate the biological behaviours of stem cells in biological systems.6
MRI is one of the most highly useful and broadly applicable imaging methods for clinical diagnosis and therapy monitoring,7 and it can noninvasively provide molecular and anatomical information about tissues.8,9 The advantages of MRI, such as no radiation exposure, rapid acquisition of images, and high temporal/spatial resolution, make it a great potential method for stem cell tracking in live subjects. However, due to the intrinsic low sensitivity of MRI, it is difficult to precisely acquire information on biological activities at a cellular or molecular level under disease conditions.10 Therefore, contrast agents have been developed to provoke signals for MRI by altering the relaxation time of water protons in tissues. Currently, most clinically available contrast agents are Gd(III) chelates for T1-weighted MR imaging and superparamagnetic iron oxide (SPIO) nanoparticles for T2-weighted MR imaging.11–14 The T1-weighted contrast agents (e.g., gadoterate and gadodiamide) can effectively shorten the longitudinal relaxation time (T1) to give brighter contrast signals, while the T2-weighted contrast agents (e.g., Feridex) can effectively shorten the transverse proton relaxation time (T2) to exhibit darker contrast signals.6,15,16
The noninvasive tracking of stem cells in live subjects requires cells to be labeled with a sufficient amount of contrast agent to enable imaging of the labeled cells under normal and disease conditions by MRI.17–19 However, the negative contrast enhancement from SPIO can be easily mistaken for a hemorrhage or blood clot. Therefore positive contrast enhancement is prefered.19 However, current clinically available Gd(III) chelates are generally hydrophilic, and so it is difficult for them to enter cells for cell labeling.20 Considering that MRI contrast agents with dual T1- and T2-weighted imaging functions can highly increase diagnostic accuracy,21 it is strongly desirable to integrate the advantages of both T1- and T2-weighted contrast agents into one system for precise MR imaging to track cells in live subjects.22
Herein, we developed gadolinium hybrid iron oxide (GdIO) nanocomposites with both T1- and T2-weighted contrast enhancement functions to label cells and then track them in biological systems by MRI. The magnetic properties of the GdIO-labeled cells were characterized and their signal intensities were also measured with MRI. Then, we systematically evaluated the potential toxicity of GdIO by treating MSCs for safety evaluation. Finally, the GdIO nanocomposites were injected into adult mouse lateral ventricles to label brain cells for MRI tracking. With the GdIO nanocomposites, the labeled cells could be precisely monitored by MRI with both T1- and T2-weighted imaging modalities, demonstrating the potential applications of using GdIO nanocomposites to label cells for tissue engineering and regenerative medicine.
Experimental
Materials
Oleic acid (>90%) and 1-octadecene (>90%) were purchased from Acros Organics (Belgium). Gadolinium(III) chloride hexahydrate (99.99%) and methyl acrylate (99%, stable with ca. 15 ppm 4-methoxyphenoland) were purchased from Alfa Aesar (USA). Sodium oleate, iron chlorides and other chemical reagents were purchased from Sinopharm Chemical Reagent Co. Ltd (China). The tetrazolium dye 3-(4,5-dimethylthiazol-2)-2,5-diphenyl-2H-tetrazolium bromide (MTT), rabbit polyclonal to polysialylated neural cell adhesion molecule (PSA-NCAM, a neuronal progenitor marker) and potassium ferrocyanide were purchased from Sigma-Aldrich Corporation (USA). All chemicals were used as received without further purification.
Dulbecco's modified eagle medium (DMEM), and fetal bovine serum (FBS) were purchased from Cyagen Biosciences (USA). Cells were maintained with DMEM containing 10% FBS and incubated in a humidity incubator at 37 °C with 5% CO2. Female Balb/c nude mice (∼6–8 week old, 18–20 g) were purchased from Charles River Laboratories (China). All the animal experiments were performed in accordance with protocol #120774 approved by the Institutional Animal Care and Use Committee (IACUC).
Preparation of GdIO nanocomposites
The GdIO nanoparticles were synthesized following a previously reported procedure.21 Briefly, iron oleate, gadolinium oleate and oleic acid were mixed in 1-octadecene under stirring. Then, the solution was heated to reflux for 2 h under the protection of an inert atmosphere. After cooling to room temperature, isopropanol was added to the solution to precipitate the nanoparticles. Biodegradable stearic acid-modified polyethyleneimine (stPEI) polymers were applied to make the GdIO nanoparticles water dispersible.13
Characterizations of GdIO nanocomposites
The morphology of the GdIO nanocomposites was investigated by transmission electron microscopy (TEM) using a JEM-2100 (JEOL, Japan) operated with an accelerating voltage of 200 kV. The magnetic hysteresis loop was measured with a Quantum Design MPMS-XL-7 system (Quantum Design, USA) at 300 K. The size and monodispersity were measured by dynamic light scattering (DLS), while the ζ-potential of the nanocomposites in phosphate buffer (pH 7.4) was checked with a Zetasizer (Malvern Zetasizer Nano system) at 25 °C. The T1- and T2-weighted MRI images and T1/T2-weighted relaxation time were measured using a 0.5 T NMR120-Analyst NMR Analyzing & Imaging system (Niumag Corporation, China). In vivo MR images were measured with a 9.4 T MRI machine (Bruker, Germany).
Labeling stem cells with GdIO nanocomposites
The MSCs were maintained in DMEM supplied with 10% FBS, and the cells were used for experiments after 3–5 passages. The MSCs were then incubated with a medium containing GdIO nanocomposites at concentrations of 1, 4, 8, 12 and 16 μg mL−1 based on Fe for 1, 3, 6, 12 and 24 h, respectively. After cell labeling, the MSCs were characterized by Prussian blue staining and the amount of Fe was analyzed.
Characterization of cell viability and labeling efficiency
To test the cell viability, the MSCs were suspended in DMEM, seeded in 96-well plates (5000 cells per well) and incubated under 5% CO2 at 37 °C for 24 h. Then, the cells were exposed to the GdIO nanocomposites at different concentrations, incubated for 72 h, and then cell viability was measured using an MTT assay.23 For apoptosis studies, the GdIO-labeled cells and untreated controls were placed on glass cover slips and stained with Hoechst to analyze the apoptotic rate of the cells.24,25 Cells exhibiting condensed chromatin were determined to be apoptotic. The average ratio of apoptotic cells was counted from more than five observation fields (100 cells per observation field) of each sample by microscopic observation. The existence of GdIO in the cells was also confirmed by Prussian blue staining. All of the samples were observed with a microscope (BZ-X700, Keyence Corp., Japan).
MR imaging of GdIO-labeled MSCs
Cell labeling was confirmed by MR imaging of the GdIO-labeled MSCs. Specifically, the MSCs (1 × 105 cells) were exposed to GdIO nanocomposites at different doses for 24 h, and then washed with PBS to be resuspended in DMEM containing 1% agarose gel. Then, the labeled MSCs were measured by MRI.26 The relaxation time of each sample was also obtained using MRI, while unlabeled cells were used as the control. Additionally, GdIO-labeled cells in animal models were imaged using a 9.4 T MRI machine. Briefly, 5 μL of GdIO (5 μg Fe) was injected into the lateral ventricles of mice and the mice were subjected to T1- and T2-weighted MR imaging. For MRI imaging, a TurboRARE sequence with the following parameters was applied: TR/TE = 2500/33 ms (T2), TR/TE = 300/10.5 ms (T1), FOV 25 × 25 mm2, slice thickness 1 mm, number of slices 3, 256 × 256 matrices, average = 4.
Histology study
After the MRI study, the mice were anesthetized with a dose of 10% chloral hydrate (0.004 mL g−1) intraperitoneally and perfused transcardially with 10% formaldehyde. Their brains were then removed, fixed and embedded in paraffin. Tissue slices from each brain were treated using Prussian blue staining to identify the brain cells labeled with the GdIO nanocomposites.27 Frozen brain tissue was cryosectioned into 10 μm thick slices and incubated for 1 h with primary antibodies in a humidified chamber. These were then washed and incubated for 45 min with secondary antibodies.28 The control was slices incubated with secondary antibodies without the presence of primary antibodies.
Results and discussion
Characterization of GdIO nanocomposites
GdIO nanocrystals were successfully obtained and their morphology was characterized by TEM. The TEM images showed that the GdIO nanocrystals were monodisperse with an average diameter of around 13.1 ± 2.5 nm (Fig. 1a). To achieve biocompatibility, the GdIO nanocrystals were then coated with amphiphilic stearic acid-polyethylenimine (stPEI) on their surfaces. After coating with the polymer, the hydrophobic–hydrophobic interaction between oleic acid in the GdIO nanocrystals and the stearic acid motifs (stPEI) led to the formation of GdIO nanoaggregates with an average diameter of approximately 82.4 ± 10.3 nm, as confirmed by TEM imaging (Fig. 1b). Zeta potential results showed that the GdIO nanocomposites displayed positive charges of around +30 mV, which is helpful for cell uptake. According to the field-dependent magnetization (M–H) curves, the GdIO nanocomposites exhibited both superparamagnetic and paramagnetic properties with unsaturated magnetic moments of 33.5 emu g−1 at a field of up to 5 T (Fig. 1c). These results indicate that the GdIO nanocomposites have a high potential for dual T1- and T2-weighted MR imaging. Then, the hydrodynamic diameter of the GdIO nanocomposites in buffers at pH 6.0 to 8.0 was measured by DLS, showing that their hydrodynamic diameter almost did not change (120–150 nm) (Fig. 1d). Furthermore, the r1 and r2 relaxivities, and MR images of the GdIO nanocomposites were evaluated. The GdIO samples embedded in 1% agarose exhibited high contrast enhancements for both T1- and T2-weighted MR imaging, displaying an average r1 value of 70.10 ± 3.65 mM−1 s−1 based on Gd and an average r2 value of 173.55 ± 6.48 mM−1 s−1 based on Fe. These results demonstrate that the GdIO nanocomposites have a high contrast ability for both T1- and T2-weighted MR imaging.
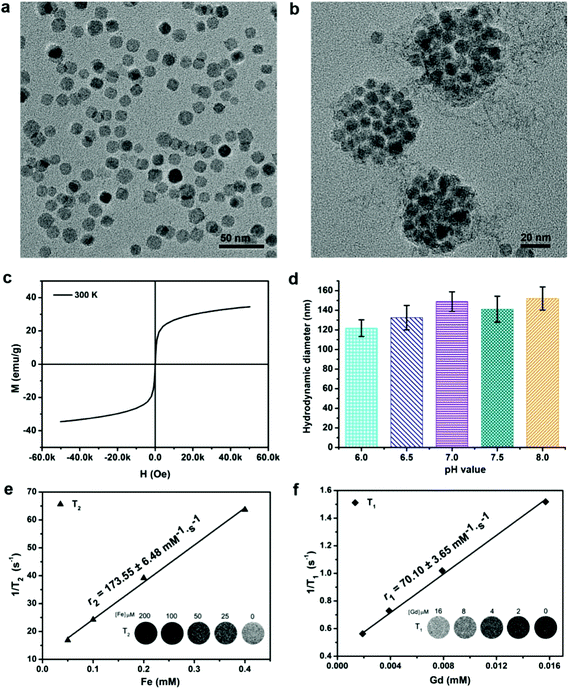 |
| Fig. 1 Characterization of the GdIO nanocomposites. (a, b) TEM images of GdIO nanocrystals (a) and GdIO nanocomposites (b); (c) the magnetic hysteresis loop of the GdIO nanocomposites at 300 K in a magnetic field of up to 5 T; (d) the average hydrodynamic diameters of the GdIO nanocomposites at pH 6.0 to 8.0, showing good stability. (e, f) T2- and T1-weighted molecular relaxivities (i.e., r1 and r2) and MR images of the GdIO nanocomposites dispersed in 1% agarose. The r1 and r2 values were calculated from the slopes of relaxivity based on the concentrations of Gd and Fe, respectively. | |
Stem cell labeling and intracellular iron amount
To determine the ideal dose and incubation time for stem cell labeling, the MSCs were treated with the GdIO nanocomposites at the concentrations of 1, 4, 8, 12 and 16 μg mL−1 based on Fe for 24 h. Meanwhile, the stem cells were also treated for 1, 3, 6, 12 and 24 h with the same concentration of 8 μg mL−1 of the GdIO nanocomposites, based on Fe. After incubation, the cells were treated by Prussian blue staining to distinguish the labeled cells from the unlabeled cells. Accordingly, the cell labeling ratio was highly dependent on the incubation time and dose of the GdIO nanocomposites (Fig. 2a and b). Almost 100% of the stem cells were labeled when exposed to nanocomposites of 16 μg mL−1 based on Fe for 24 h (Fig. 2c and d). This also demonstrates that the MSCs could be labeled in a facile way by just adding the GdIO nanocomposites to cell culture media and incubating for 24 h. The labeling of the MSCs was further confirmed by cellular TEM, the results of which strongly support the fact that the GdIO nanocomposites were located inside the intracellular space of MSCs, but outside of the cell nuclei (Fig. 2e). Atomic absorption spectroscopy of the labeled MSCs also demonstrated that a higher amount of Fe was inside of the stem cells when exposed to a higher dose of the nanocomposites (Fig. 2f), confirming that the cellular uptake of the GdIO nanocomposites was dose-dependent.
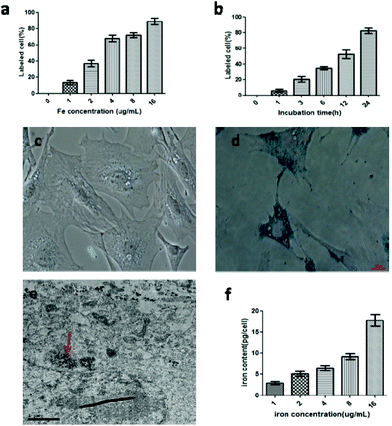 |
| Fig. 2 Characterization of MSCs labeled with the GdIO nanocomposites. (a, b) The cell labeling ratio of MSCs treated with nanocomposites at the concentrations of 1, 4, 8, 12 and 16 μg mL−1 based on Fe for 24 h (a); and treated with nanocomposites at the concentration of 8 μg mL−1 based on Fe for 1, 3, 6, 12 and 24 h (b); Prussian blue staining of unlabeled MSCs (c) and MSCs treated with GdIO (16 μg mL−1 Fe) for 24 h (d). The morphology of these labeled MSCs was not changed, while both the blue color from the Prussian blue staining of Fe and the TEM image of the cells labeled with GdIO (arrows) demonstrate that the nanocomposites were located in the cytoplasm of the MSCs (e). Scale bar is 1 μm. (f) The intracellular level of Fe in the MSCs after treating with the GdIO nanocomposites, demonstrating the cellular uptake of the nanocomposites was highly dependent on the labeling dose. | |
Cytotoxicity and differentiation
It is possible to label MSCs with sufficient amounts of the nanocomposites for MRI without affecting their viability and proliferation. We next examined the cytotoxicity of the GdIO nanocomposites with the above cell labeling concentrations. According to the results of an MTT assay (Fig. 3a), the viabilities of the MSCs after exposure to the nanocomposites were similar to that of control cells. These results demonstrate that it is safe to label MSCs with GdIO nanocomposites using the above doses. Besides, it is better to acquire images with the lowest amount of the nanocomposites for cell labeling. In this study, we chose to label the stem cells with GdIO nanocomposites at the concentration of 16 μg mL−1 based on Fe and then incubate them for 24 h. Hoechst staining revealed that there was no apoptosis caused by the GdIO nanocomposite labeling (Fig. 3b and c). Furthermore, the expression of glycosaminoglycan (GAG), a marker for chondrogenic potential, was measured to investigate if there was any difference between the GdIO-labeled and unlabeled MSCs during chondrogenic differentiation. The GAG-rich extracellular matrix of both the GdIO-labeled and untreated MSCs was stained using Safranin O (Fig. 3d and e) and toluidine blue (Fig. 3f and g), indicating that the chondrogenic differentiation of the MSCs was not affected by labeling with the GdIO nanocomposites.
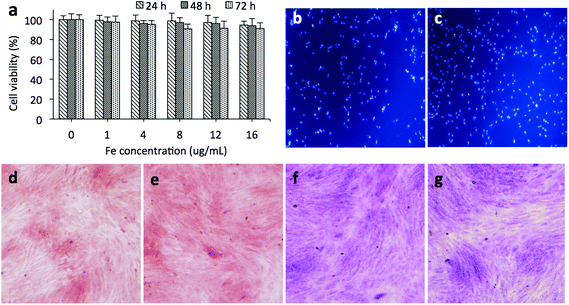 |
| Fig. 3 Biocompatibility assessment of the GdIO nanocomposites. (a) The cytotoxicity of the GdIO nanocomposites against MSCs measured using an MTT assay. No statistical significance was found compared to the control. (b, c) Hoechst staining of the GdIO-labeled (b) and unlabeled MSCs (c), revealing there was no statistical significance between the two groups. Safranin O (d, e) and toluidine blue staining (f, g) showed that there was no obvious difference in chondrogenic differentiating capacity between the unlabeled (d, f) and GdIO-labeled MSCs (e, g). | |
In vitro MR imaging of GdIO-labeled MSCs
The high cellular uptake of the GdIO nanocomposites together with their high relaxivity make them suitable for labeling and tracking MSCs by MRI. To confirm the contrast enhancement, the GdIO-labeled MSCs were washed and resuspended in 1% agarose gel for MR imaging. The T1- and T2-weighted MR images of the GdIO-labeled cells were obtained using a 0.5 T NMR120-Analyst NMR Analyzing & Imaging system for T1-weighted imaging and a 9.4 T MR scanner (Bruker 94/20, Germany) for T2-weighted imaging. Both the T1- and T2 relaxation times decreased in association with the increased incubation concentration from 0 to 16 μg mL−1 based on Fe (Fig. 4a and c), suggesting that the GdIO-labeled cells could be easily tracked by MRI. Similar results were also observed with different labeling times (0, 3, 6, 12 and 24 h), with shorter relaxation times observed for cells after incubating with GdIO nanocomposites for longer periods (Fig. 4b and d). To compare the contrast enhancement, both the T1- and T2-weighted MR images of the GdIO-labeled MSCs were also obtained after labeling for different incubation times and at different doses. When the incubation dose and time with the GdIO nanocomposites were increased, the contrast signals were reduced in the T2-weighted MR images and increased in the T1-weighted MR images. This indicates that the GdIO nanocomposites can be applied for dual-modal MR imaging in cell labeling and tracking.
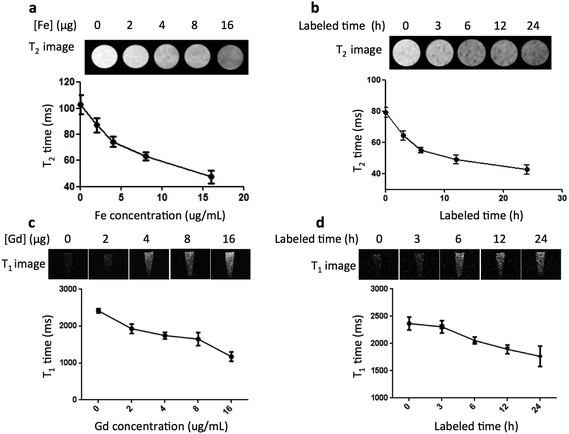 |
| Fig. 4 MR images of GdIO-labeled MSCs. (a, b) T2-weighted MR images and relaxation times of MSCs treated with GdIO nanocomposites at different concentrations and for different incubation times. (c, d) T1-weighted MR images and relaxation times of MSCs treated with GdIO nanocomposites at different concentrations and for different incubation times. The data are expressed as the mean ± SD (n = 5). | |
In vivo MR imaging of GdIO nanocomposites
Considering that the GdIO nanocomposites can directly label MSCs in vitro, they were further applied to label mouse neuronal progenitor cells in vivo. The GdIO nanocomposites (5 μg Fe) were injected into the right lateral ventricle anterior of mice. Then, the mice were scanned using a 9.4 T MRI machine. After administration of the GdIO nanocomposites for 24 h, both T1- and T2-weighted contrast enhancements were found at the lateral ventricles (Fig. 5a–d). After injection for 72 h, the brain tissues were harvested and the presence of iron oxide in the brain cells was confirmed by Prussian blue staining (Fig. 5e). Interestingly, the blue colors from the Prussian blue staining of iron oxide were found in the brain tissues, demonstrating that the GdIO nanocomposites are able to infiltrate from the anterior lateral ventricle sites into the brain tissues and translocate into neurons. In the same histological sections, we observed numerous PSA-NCAM positive cells (mouse neuronal progenitors) (Fig. 5f), indicating that GdIO could be applied for in situ neuro-progenitor cell labeling and that endogenous neuroblast migration could be traced by MRI.28 Despite the absence of obvious in vitro toxicity of GdIO to MSCs, its potential in vivo toxicity is still a challenge to be addressed. We supervised the behaviors of the mice after MR imaging and no obvious signs of acute toxic effects, such as abnormalities in body weight, eating, drinking, activity, urination or neurological status were noticed for those treated mice. However, more careful studies are still required to systematically examine the potential long-term toxicity of GdIO. Furthermore, GdIO labels the neuro-progenitor cells non-specifically and we are now working on the surface engineering of GdIO with targeting motifs for the design of a more effective labeling and tracking strategy.
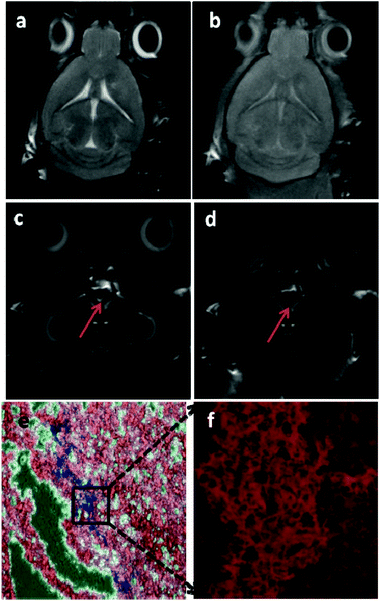 |
| Fig. 5
In vivo MR images of mice brains with cells labeled with the GdIO nanocomposites. T2-weighted MR images (a, c) and T1-weighted MR images (b, d) of mice lateral ventricles post injection of the GdIO nanocomposites. (e) Prussian blue staining of brain tissues at 72 h after injection of the GdIO nanocomposites. The cells with iron were stained with a blue color by the Prussian blue staining, and numerous PSA-NCAM positive cells (mouse neuronal progenitors) (dark pink color) (f) were observed in the same histological sections, indicating that GdIO could be applied for in situ neuro-progenitor cell labeling and tracking by MRI. | |
Conclusions
In this work, we have developed a novel dual T1- and T2-weighted MRI contrast agent from GdIO nanocomposites, which can easily be internalized by cells as tested in vitro and in vivo, without influencing the cell viability. The nanocomposites demonstrated high contrast enhancements in media for T1- and T2-weighted MR imaging, and the labeled MSCs also could boost high contrast signals for investigations by MRI, demonstrating the potential applications of the nanocomposites as cellular MRI contrast agents. The dual-functions of the GdIO nanocomposites for enhancing T1- and T2-weighted MR signals in vitro and in vivo mean they have a high potential to be further applied in clinical applications. Furthermore, the facile preparation route together with the biocompatible surface coating strategy could be utilized to design other functional contrast agents and theranostic systems for imaging or imaging-guided therapy.
Acknowledgements
This work was supported by the Major State Basic Research Development Program of China (973 Program) (2013CB733802 and 2014CB744503); the National Nature Science of Foundation of China (NSFC) (51273165, 81422023, and U1505221); the Key Project of Chinese Ministry of Education (212149); the Fundamental Research Funds for the Central Universities (20720160065 and 20720150141); and the Program for New Century Excellent Talents in University (NCET-13-0502).
References
-
N. Beyer Nardi and L. da Silva Meirelles, Handbook of experimental pharmacology, 2006, pp. 249–282 Search PubMed.
- Y. Wu, B. Zhou, F. Xu, X. Wang, G. Liu, L. Zheng, J. Zhao and X. Zhang, Acta Biomater., 2016, 46, 165–176 CrossRef CAS PubMed.
- H. S. Hong, Y. H. Kim and Y. Son, Arch. Pharmacal Res., 2012, 35, 201–211 CrossRef CAS PubMed.
- Y. J. Chuah, S. Kuddannaya, M. H. Lee, Y. Zhang and Y. Kang, Biomater. Sci., 2015, 3, 383–390 RSC.
- J. Ozsvar, S. M. Mithieux, R. Wang and A. S. Weiss, Biomater. Sci., 2015, 3, 800–809 RSC.
- G. Liu, Z. Wang, J. Lu, C. Xia, F. Gao, Q. Gong, B. Song, X. Zhao, X. Shuai, X. Chen, H. Ai and Z. Gu, Biomaterials, 2011, 32, 528–537 CrossRef CAS PubMed.
- R. Weissleder and U. Mahmood, Radiology, 2001, 219, 316–333 CrossRef CAS PubMed.
- J. W. Bulte and D. L. Kraitchman, NMR Biomed., 2004, 17, 484–499 CrossRef CAS PubMed.
- C. Yang, R. Tian, T. Liu and G. Liu, Molecules, 2016, 21 CAS.
- T. F. Massoud and S. S. Gambhir, Genes Dev., 2003, 17, 545–580 CrossRef CAS PubMed.
- C. Billotey, C. Aspord, O. Beuf, E. Piaggio, F. Gazeau, M. F. Janier and C. Thivolet, Radiology, 2005, 236, 579–587 CrossRef PubMed.
- R. Hao, R. Xing, Z. Xu, Y. Hou, S. Gao and S. Sun, Adv. Biomater., 2010, 22, 2729–2742 CAS.
- C. He, S. Jiang, H. Jin, S. Chen, G. Lin, H. Yao, X. Wang, P. Mi, Z. Ji, Y. Lin, Z. Lin and G. Liu, Biomaterials, 2016, 83, 102–114 CrossRef CAS PubMed.
- U. Sakulkhu, M. Mahmoudi, L. Maurizi, G. Coullerez, M. Hofmann-Amtenbrink, M. Vries, M. Motazacker, F. Rezaee and H. Hofmann, Biomater. Sci., 2015, 3, 265–278 RSC.
- Z. Wang, G. Liu, J. Sun, B. Wu, Q. Gong, B. Song, H. Ai and Z. Gu, J. Nanosci. Nanotechnol., 2009, 9, 378–385 CrossRef CAS PubMed.
- H. Liu, J. Zhang, X. Chen, X. S. Du, J. L. Zhang, G. Liu and W. G. Zhang, Nanoscale, 2016, 8, 7808–7826 RSC.
- L. Josephson, C. H. Tung, A. Moore and R. Weissleder, Bioconjugate Chem., 1999, 10, 186–191 CrossRef CAS PubMed.
- M. Barrow, A. Taylor, D. J. Nieves, L. K. Bogart, P. Mandal, C. M. Collins, L. R. Moore, J. J. Chalmers, R. Levy, S. R. Williams, P. Murray, M. J. Rosseinsky and D. J. Adams, Biomater. Sci., 2015, 3, 608–616 RSC.
- R. Weissleder, M. Nahrendorf and M. J. Pittet, Nat. Mater., 2014, 13, 125–138 CrossRef CAS PubMed.
- P. Caravan, J. J. Ellison, T. J. McMurry and R. B. Lauffer, Chem. Rev., 1999, 99, 2293–2352 CrossRef CAS PubMed.
- Z. Zhou, D. Huang, J. Bao, Q. Chen, G. Liu, Z. Chen, X. Chen and J. Gao, Adv. Mater., 2012, 24, 6223–6228 CrossRef CAS PubMed.
- L. E. Jennings and N. J. Long, Chem. Commun., 2009, 3511–3524, 10.1039/b821903f.
- Z. Y. Wang, G. Liu and J. Y. Sun,
et al.
, J. Nanosci. Nanotechnol., 2008, 8, 1–8 CrossRef.
- Z. H. Qu, X. L. Zhang, T. T. Tang and K. R. Dai, Biochem. Biophys. Res. Commun., 2008, 370, 332–337 CrossRef CAS PubMed.
- G. Liu, J. Tian, C. Liu, H. Ai, Z. Gu, J. Gou and X. Mo, J. Mater. Res., 2009, 24, 1317–1321 CrossRef CAS.
- M. H. El-Dakdouki, K. El-Boubbou, D. C. Zhu and X. Huang, RSC Adv., 2011, 1, 1449–1452 RSC.
- M. R. Loebinger, P. G. Kyrtatos, M. Turmaine, A. N. Price, Q. Pankhurst, M. F. Lythgoe and S. M. Janes, Cancer Res., 2009, 69, 8862–8867 CrossRef CAS PubMed.
- B. Iordanova and E. T. Ahrens, NeuroImage, 2012, 59, 1004–1012 CrossRef CAS PubMed.
Footnote |
† These authors contributed equally. |
|
This journal is © The Royal Society of Chemistry 2017 |
Click here to see how this site uses Cookies. View our privacy policy here.