DOI:
10.1039/C6QM00190D
(Research Article)
Mater. Chem. Front., 2017,
1, 867-872
Facile construction of butadiynylene based conjugated porous polymers by cost-effective Glaser coupling†
Received
26th August 2016
, Accepted 17th October 2016
First published on 23rd November 2016
Abstract
A series of butadiynylene (BD) based conjugated microporous polymers (CMPs) were facilely synthesized using various aromatic alkyne monomers with different geometries via a Glaser coupling reaction under mild conditions. All these CMPs form gels in situ after self-polycondensation. The chemical structures, morphologies, surface areas, CO2 and CH4 uptake capacities of the freeze-dried gels were thoroughly characterized. All four CMPs have large specific surface areas (up to 1008 m2 g−1), big total pore volumes (up to 1.11 cm3 g−1), high CO2 uptake capacities (up to 3.78 mmol g−1) and high CH4 adsorption capacities (up to 0.95 mmol g−1). Bearing densely incorporated active sites (C
C bonds) for the binding of metallic ions (e.g. Li+), excellent porosity, and a conjugated network structure, these BD-CMPs hold great promise for applications in gas adsorption, heavy metal accumulation, heterogeneous catalysis, lithium batteries, etc.
Introduction
Conjugated microporous polymers (CMPs) are a new kind of functional polymer system, with rigid and fully π-conjugated skeletons, permanent microporosity and apparent specific surface areas.1 Since the first example of poly(aryleneethynylene) (PAE) CMPs reported by the Cooper group in 2007,2 tremendous efforts have been devoted to develop new CMPs with various functionalities. For example, CMPs hold great promise for applications in gas storage/separation,3 heterogeneous catalysis,4 chemical- and bio-sensing,5 light emission,6 photocatalysis,7 energy storage and conversion,8etc. Thus, the exploration of the synthesis and function of novel CMPs is a burgeoning new research area in the field of organic porous materials. However, most of the reported CMPs (e.g. PAEs) suffer from noble metal catalysed copolymerization of two or more monomers,9d which always require multi-step synthesis.9 Furthermore, most of the reported CMPs with high surface areas are insoluble powders and are often subjected to harsh reaction conditions to ensure the evolution of CMP networks that help to achieve high porosity.10 Thus, exploring a facile method to construct processable porous CMP networks under mild conditions in a cost-effective manner is required and remains challenging. Recently, a few examples of diynylene based CMP gels were developed via Pd(II)/Cu(I) co-catalysed homo-coupling of terminal alkynes.11 These CMP xerogels possess outstanding elastic properties, and can be organized into the desired modules.11b Herein, we reported a facile synthesis of four butadiynylene (BD) based CMPs using cost-effective CuCl catalysed Glaser coupling reactions under mild conditions.12 All the BD-CMPs form stable physical gels in situ upon self-polycondensation. The BD-CMP freeze-dried gels have large specific surface areas and big pore volumes with hierarchical pore structures and exhibit superior performances for CO2 and CH4 capture. Our results demonstrate a convenient and highly efficient method of weaving various simple and sole monomers with tunable structures and properties to generate processable CMP gels. The solvated CMP gels could be easily transformed to a xerogel monolith upon demand for further applications.
For comparison and investigation of the structure–property relationship of the butadiynylene (BD) based CMPs, four different BD-CMPs were synthesized. Four terminal alkyne monomers, namely 1,3,5-tris-(4-ethynylphenyl)benzene (TPB), tri(4-ethynylphenyl)amine (TPA), tetrakis(4-ethynylphenyl)methane (TPM), and 2,2′,7,7′-tetraethynyl-9,9′-spirobi[fluorene] (SPF) were selected and polymerized to afford TPB-BD-CMP, TPA-BD-CMP, TPM-BD-CMP, and SPF-BD-CMP, respectively (Fig. 1a). The in situ formed gels were freeze-dried into xerogels after the solvent was exchanged with water, and the xerogels were thoroughly characterized using infrared spectroscopy (IR), UV-vis diffuse reflectance spectroscopy, solid-state NMR, thermal gravimetric analysis (TGA), powder X-ray diffraction (PXRD), field emission scanning electron microscopy (FE-SEM), transmission electron microscopy (TEM), BET surface area analysis, etc.
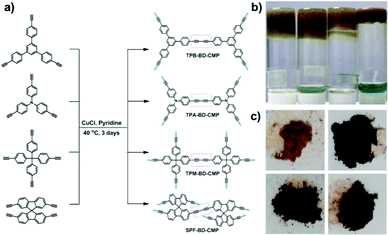 |
| Fig. 1 (a) Synthetic routes for the four BD-CMPs; (b) the picture of the BD-CMP gels (from left to right are: TPB-BD-CMP, TPA-BD-CMP, TPM-BD-CMP and SPF-BD-CMP); and (c) the lyophilized xerogels. | |
Experimental
Materials
Trimethylsilylacetylene and p-bromoacetophenone were purchased from Acros, tetraphenylmethane was purchased from Alfa Aesar, and triphenylamine was obtained from Sigma Aldrich. All chemicals and reagents were used directly without further purification. Solvents were purified according to standard procedures. All the alkynyl monomers were synthesized using modified procedures from the literature (see the ESI†).
Physical measurements
FT-IR spectra were collected in the transmission mode on a Bruker Alpha spectrometer using KBr pellets with a scan range of 400–4000 cm−1. The UV-vis diffuse reflectance spectra were obtained from a PerkinElmer Lambda 750 spectrophotometer using standard procedures. The 1H NMR spectra of all the organic compounds were recorded on a Bruker AVANCE III-400 NMR spectrometer. Solid-state NMR experiments were performed on a Bruker WB AVANCE II 400 MHz NMR spectrometer. The 13C cross-polarization magic angle spinning (CP/MAS) spectra were recorded with a 4 mm double-resonance MAS probe and at a MAS rate of 10.0 kHz with a contact time of 2 ms (ramp 100) and a pulse delay of 3 s. The residual Cu content was determined via ICP-MS techniques using the Agilent 7700X ICP-MS model. The samples were prepared via nitrohydrochloric acid digestion before measurement. The thermal properties of all the CMPs were evaluated using thermogravimetric analysis (TGA) with a differential thermal analysis instrument (NETZSCH STA 409PC analyzer) in the temperature range from 30 °C to 700 °C under an air atmosphere with a heating rate of 15 °C min−1. Powder X-ray diffraction experiments were carried out on a X-ray diffractometer model Flex 600 diffractometer (Japan) at 40 kV, 15 mA with a Cu-target tube and a graphite monochromator. Field emission scanning electron microscopy (FE-SEM) was performed on a Hitachi Limited model S-4800 microscope operating at an accelerating voltage of 3.0 kV. High resolution transmission electron microscopy (HR-TEM) was performed on a JEOL model JEM-2100F microscope. The nitrogen and carbon dioxide adsorption and desorption isotherms were measured at 77 K and 273 K using a Bel Japan Inc. model BELSOPR-mini II analyser, respectively. The CH4 adsorption/desorption isotherms were recorded at 273 K using a Micromeritics ASAP 2020M gas adsorption analyser. The samples were degassed at 150 °C for 3 h under vacuum (10−5 bar) before analysis. The surface areas were calculated from the nitrogen adsorption data. The pore size distribution was calculated from the nitrogen adsorption branch with the nonlocal density functional theory (NLDFT).
Results and discussion
The synthetic routes towards the four butadiynylene based CMPs (BD-CMPs) are shown in Fig. 1a. The detailed synthetic procedures of four alkynyl monomers namely 1,3,5-tris-(4-ethynylphenyl)benzene,13 tri(4-ethynylphenyl)amine,14 tetrakis(4-ethynylphenyl)methane,15 and 2,2′,7,7′-tetraethynyl-9,9′-spirobi[fluorene]16 are summarized in the ESI.† The synthesis of the designed polymer networks were modified according to a reported procedure.12 In general, CuCl was added to the solutions of alkynyl monomers in pyridine, which then remained, unstirred, at 40 °C for 3 days. Usually, the reaction mixture started gelation after heating for around two days. The in situ formed gels (Fig. 1b) were exchanged with water thoroughly to remove pyridine. The residual Cu content in the BD-CMPs is negligibly low at around 0.10–0.24%, as revealed by the inductively coupled plasma mass spectrometry (ICP-MS) measurements. The gels were freeze-dried to xerogels (Fig. 1c) for further characterization. A more systematic study on the influence of synthetic conditions on the polymer networks is given in the ESI† (Table S2 and Fig. S6). The formation of a diynyl linkage was verified by the solid-state 1H-13C cross-polarization magic-angle spinning (CP/MAS) NMR spectrum recorded at a MAS rate of 10 kHz and a CP contact time of 2 ms. The signal assignment of carbons in the CMP skeletons is illustrated in Fig. 2. Two characteristic peaks of the sp carbons appeared at 82.3, 76.7 ppm for TPB-BD-CMP; 82.3, 76.0 ppm for TPA-BD-CMP; 83.0, 76.2 ppm for TPM-BD-CMP and 83.0, 76.0 ppm for SPF-BD-CMP, respectively. These results suggest the successful formation of the diynyl linkage in the CMP skeletons. The most intensive signals of the NMR spectra (i.e. 142.0, 132.5, 127.2, 122.1 ppm for TPB-BD-CMP; 147.3, 133.0, 130.1, 118.9 ppm for TPA-BD-CMP; 146.8, 130.8, 121.4 ppm for TPM-BD-CMP and 148.8, 142.0, 129.8, 122.3 ppm for SPF-BD-CMP) correspond to the phenyl carbons (Fig. 2). While in the case of TPM-BD-CMP, an additional signal of δ = 65.3 ppm can be detected in the up-field which is assignable to the central sp3 carbon of the tetraphenylmethane core. Similarly, the sp3 carbon signal of the spirobifluorene core in SPF-BD-CMP was observed in δ = 66.0 ppm. The successful formation of the diynyl linkage was further confirmed through Fourier-transform infrared (FT-IR) spectra analysis. As displayed in Fig. S1 (ESI†), the apparent stretching vibrations (
C–H st) around 3300 cm−1 for the alkyne monomers (red line) were nearly disappeared in the BD-CMPs. Stretching vibrations appearing around 2110 cm−1 are assignable to the triple bonds (C
C st) from the terminal alkyne monomers blue-shifted to ca. 2180 cm−1 in the diynyl linkage of the BD-CMPs. Consequently, these two characteristic stretching vibration peaks indicate the successful formation of the diynyl linkage in high efficiency.
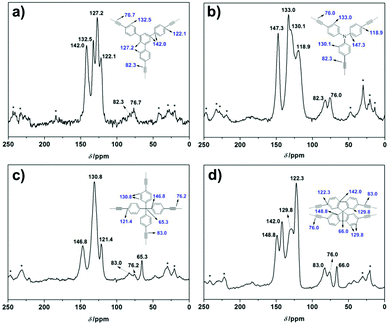 |
| Fig. 2 Solid-state 1H-13C CP/MAS NMR spectra of the four BD-CMPs recorded at a MAS rate of 10 kHz and a contact time of 2 ms. Asterisks denote spinning sidebands. | |
The high polymerization degree of the diynyl based CMPs could also be supported by their excellent thermal stabilities. As displayed in Fig. S3 (ESI†), all four BD-CMPs are stable up to 330 °C under an air atmosphere. The weight loss of 5% for TPB-BD-CMP, TPA-BD-CMP, TPM-BD-CMP and SPF-BD-CMP was observed at 348 °C, 334 °C, 372 °C and 342 °C, respectively. Moreover, all four BD-CMPs also exhibited good hydrothermal stabilities (Fig. S2, ESI†). All CMPs were obtained as amorphous powders without crystallinity as confirmed by powder X-ray diffraction analysis. No distinctive diffraction peaks were detected (Fig. S4, ESI†), as reported for most of the other reported CMP networks.
To investigate the conjugation extension effect of the BD-CMPs compared with their monomers, the UV-vis diffuse reflectance spectra of these four BD-CMPs and their alkyne monomers were recorded in the solid-state. All four CMPs exhibited broad absorption in the visible region without distinctive peaks, which is in accordance with their dark or dark brownish appearance. However, compared with their corresponding monomers, all four BD-CMP absorption edges displayed a significant redshift of more than 150 nm. These results indicate the efficient conjugation extension of the polymer network skeletons (Fig. 3).
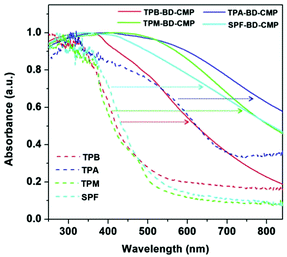 |
| Fig. 3 UV-Vis diffuse reflectance spectra of the four BD-CMPs (solid line) and their corresponding alkyne monomers (dot line). | |
The porosity of these four diynyl based CMPs was investigated using nitrogen adsorption/desorption measurements. The isotherms of the four CMPs all exhibited a steep increase in the lower P/P0 regions (<0.05, Fig. 4 and Fig. S5, ESI†) due to their microporosity. However, TPM-BD-CMP and SPF-BD-CMP exhibited another steep increase at higher P/P0 values (>0.80), which indicate the coexistence of the mesopores and macropores. The Brunauer–Emmett–Teller (BET) surface areas were evaluated to be 657, 543, 1008 and 833 m2 g−1 for TPB-BD-CMP, TPA-BD-CMP, TPM-BD-CMP and SPF-BD-CMP, respectively. The total pore volumes, estimated from the amount of gas adsorbed at P/P0 = 0.99, were 0.96, 1.11, 0.50, and 0.55 cm3 g−1, respectively. Significant desorption hysteresis loops were observed for TPB-BD-CMP, TPA-BD-CMP and SPF-BD-CMP (Fig. S4, ESI†), which may be explained by the swelling effect as a result of gas sorption. Fig. 4b shows the pore size distribution (PSD) profiles of the four CMPs as calculated by using the nonlocal density functional theory (NLDFT). The PSD profiles reveal the presence of both micropores with a size of 0.91 nm for TPB-BD-CMP, 0.59 nm, 1.31 nm for TPA-BD-CMP, 1.17 nm for TPM-BD-CMP, 1.29 nm for SPF-BD-CMP, and mesopores with a size of 2.98 nm for TPM-BD-CMP and broad shoulder macropores with a size of 75 nm for TPB-BD-CMP and 93 nm for TPA-BD-CMP. These results indicate that most of the BD-CMPs have hierarchical pore structures, which is very useful for mass transport that plays important roles in catalysis and energy related device applications.17
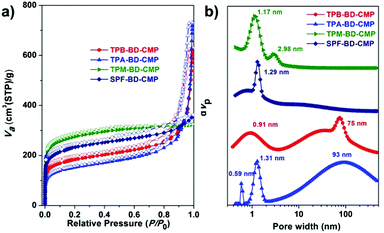 |
| Fig. 4 (a) Nitrogen adsorption/desorption isotherms at 77 K (the adsorption branches are labeled with filled symbols, and the desorption branches are labeled with empty symbols) and (b) pore size distribution curves of TPB-BD-CMP (red), TPA-BD-CMP (blue), TPM-BD-CMP (green) and SPF-BD-CMP (cyan) via NLDFT calculations. | |
The morphologies of these four CMPs were investigated using field emission scanning electron microscopy (FE-SEM) and transmission electron microscopy (TEM). The FE-SEM images of the obtained xerogels revealed different morphologies (Fig. 5). For example, TPB-BD-CMP exhibited a continuous fibril-interlocked network structure. While TPA-BD-CMP was revealed to have a fine spherical particle morphology with a particle size around 50 nm (Fig. S5b, ESI†). In contrast, TPM-BD-CMP and SPF-BD-CMP showed similar morphologies of discontinuous network structures composed of small particles. Interestingly, the macropores can be visualized using the SEM images for TPB-CMP and TPA-BD-CMP, which were consistent with the PSD results. These macropores might be introduced by the sublimation of the pre-frozen solvent during the drying process.12 Furthermore, the high-resolution TEM images for the four CMPs (Fig. 6) clearly demonstrate their intrinsic microporosity as revealed by the nitrogen adsorption isotherm. The micropores were homogenously distributed, as shown in Fig. 6.
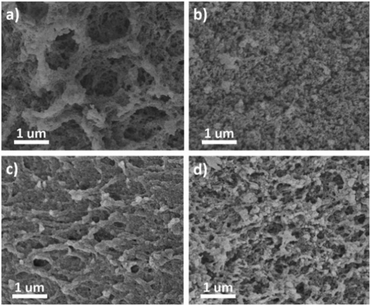 |
| Fig. 5 FE-SEM images of (a) TPB-BD-CMP, (b) TPA-BD-CMP, (c) TPM-BD-CMP and (d) SPF-BD-CMP (all the scale bars are 1 μm). | |
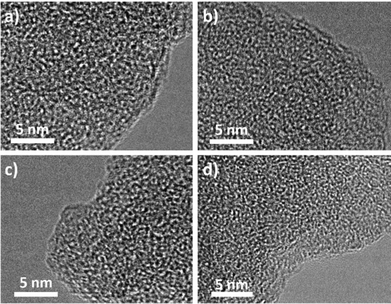 |
| Fig. 6 High resolution TEM images of the CMP samples re-dispersed in MeOH, revealing the inherent porous structures of (a) TPB-BD-CMP, (b) TPA-BD-CMP, (c) TPM-BD-CMP and (d) SPF-BD-CMP (all the scale bars are 5 nm). | |
The xerogels of these four CMPs with high surface areas are expected to show both high capacity and reversibility in gas sorption. As shown in Fig. 7, CO2 and CH4 adsorption/desorption isotherms were obtained at 273 K. CO2 uptakes for TPB-BD-CMP, TPA-BD-CMP, TPM-BD-CMP, and TPA-BD-CMP are approximately 2.51, 2.94, 3.78, and 3.25 mmol g−1 at 1 bar/273 K respectively (Fig. 7a and Fig. S9a, ESI†). The CO2 adsorption capacities generally correlate with their specific surface areas. Thus, TPM-BD-CMP and SPF-BD-CMP exhibit higher CO2 uptake than TPB-BD-CMP and TPA-BD-CMP. However, TPA-BD-CMP (Fig. 7a, blue line) displays a larger CO2 capacity than TPB-BD-CMP (Fig. 7a, red line) although it has a smaller specific surface area. This could be ascribed to the nitrogen-containing skeleton of TPA-BD-CMP, which commonly exhibited a higher CO2 affinity than non-nitrogen hydrocarbon polymers.18 On the other hand, CH4 uptakes for the TPB-BD-CMP, TPA-BD-CMP, TPM-BD-CMP, and SPF-BD-CMP are approximately 0.51, 0.59, 0.95, and 0.85 mmol g−1 at 1.2 bar/273 K respectively (Fig. 7b and Fig. S9b, ESI†), which is in accordance with the trends of CO2 adsorption. Both TPM-BD-CMP and SPF-BD-CMP have impressively high CO2 capacities and CH4 uptake abilities in comparison with most of the other reported CMPs and COFs (ESI,† Table S1). Moreover, these porous polymers conduct high gas capture performances upon Li doping at the densely entangled alkyne sites, as evidenced by recent examples.19
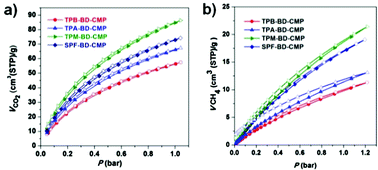 |
| Fig. 7 Volumetric (a) CO2 and (b) CH4 sorption analysis for the four BD-CMPs at 273 K (the adsorption branch is labeled with filled symbols, and the desorption branch is labeled with empty symbols). | |
Conclusions
In summary, we have synthesized a series of new butadiynyl linked CMPs via a Glaser coupling reaction under mild conditions and an air atmosphere. These butadiynyl based CMPs all formed physical gels in situ during polymerization. The dried xerogels exhibit high surface areas, large pore volumes and a hierarchical pore size distribution. Moreover, they are among some of the best organic porous polymers for CO2 capture, with a CO2 capacity of up to 3.78 mmol g−1 (i.e. 16.6 wt%). Interestingly, due to the efficient π-conjugation effect of the C
C diynyl bond, their conjugation fully extended over the network skeleton compared to their monomers, as evidenced by their colour and UV-vis diffuse reflectance spectra. Due to the high density of active sites (C
C bonds) for binding of metallic ions (e.g. Li+), we anticipate that these butadiynyl based CMPs could have potential applications in gas storage or electrode materials for lithium batteries. These research studies are currently in progress in our laboratory.
Acknowledgements
The authors are grateful to Dr Jing Mao (Tianjin University) for her help with the TEM measurements. This work was supported by the National Natural Science Foundation of China (51522303, 21602154), the National Key Basic Research Program of China (2015CB856500), the open project of the State key laboratory of supramolecular structures and materials, Jilin University (sklssm201606), Tianjin Municipal Science and Technology Commission Grant (15JCYBJC53000) and the Thousand Youth Talents Plan.
References
-
(a) A. I. Cooper, Adv. Mater., 2009, 21, 1291 CrossRef CAS;
(b) R. Dawson, A. I. Cooper and D. J. Adams, Prog. Polym. Sci., 2011, 37, 530 CrossRef;
(c) A. Thomas, Angew. Chem., Int. Ed., 2010, 49, 8328 CrossRef CAS PubMed;
(d) B. Zhou and L. Chen, Acta Chim. Sin., 2015, 73, 487 CrossRef CAS;
(e) Y. Xu, S. Jin, H. Xu, A. Nagai and D. Jiang, Chem. Soc. Rev., 2013, 42, 8012 RSC.
- J. X. Jiang, F. Su, A. Trewin, C. D. Wood, N. L. Campbell, H. Niu, C. Dickinson, A. Y. Ganin, M. J. Rosseinsky, Y. Z. Khimyak and A. I. Cooper, Angew. Chem., Int. Ed., 2007, 46, 8574 CrossRef CAS PubMed.
-
(a) U. Diaz and A. Corma, Coord. Chem. Rev., 2016, 311, 85 CrossRef CAS;
(b) Z. Xiang and D. Cao, J. Mater. Chem. A, 2013, 1, 2691 RSC;
(c) Q. Chen, M. Luo, P. Hammershøj, P. Zhou, Y. Han, B. W. Laursen, C. Yan and B. Han, J. Am. Chem. Soc., 2012, 134, 6084 CrossRef CAS PubMed;
(d) Q. Chen, M. Luo, T. Wang, J. Wang, D. Zhou, Y. Han, C. Zhang, C. Yan and B. Han, Macromolecules, 2011, 44, 5573 CrossRef CAS;
(e) W. Lu, D. Yuan, D. Zhao, C. I. Schilling, O. Plietzsch, T. Muller, S. Brase, J. Guenther, J. Bluemel and R. Krishna, Chem. Mater., 2010, 22, 5964 CrossRef CAS;
(f) T. Hasell, C. Wood, R. Clowes, J. Jones, Y. Khimyak, D. Adams and A. I. Cooper, Chem. Mater., 2010, 22, 557 CrossRef CAS;
(g) J. Xu, F. Deng, J. M. Simmons, S. Qiu and G. Zhu, Angew. Chem., Int. Ed., 2009, 48, 9457 CrossRef PubMed.
-
(a) Y. Zhang and S. N. Riduan, Chem. Soc. Rev., 2012, 41, 2083 RSC;
(b) Q. Sun, Z. Dai, X. Meng and F.-S. Xiao, Chem. Soc. Rev., 2015, 44, 6018 RSC;
(c) Y. Zhang and J. Y. Ying, ACS Catal., 2015, 5, 2681 CrossRef CAS;
(d) P. Kaur, J. T. Hupp and S. T. Nguyen, ACS Catal., 2011, 1, 819 CrossRef CAS;
(e) L. Chen, Y. Yang and D. Jiang, J. Am. Chem. Soc., 2010, 132, 9138 CrossRef CAS PubMed;
(f) L. Chen, Y. Yang, Z. Guo and D. Jiang, Adv. Mater., 2011, 23, 3149 CrossRef CAS PubMed;
(g) J. Jiang, C. Wang, A. Laybourn, T. Hasell, B. Clowes and A. I. Cooper, Angew. Chem., Int. Ed., 2011, 50, 1072 CrossRef CAS PubMed;
(h) P. Zhang, Z. Weng, J. Guo and C. Wang, Chem. Mater., 2011, 23, 5243 CrossRef CAS.
-
(a) X. Liu, Y. Xu and D. Jiang, J. Am. Chem. Soc., 2012, 134, 8738 CrossRef CAS PubMed;
(b) Y. Kou, Y. Xu, Z. Guo and D. Jiang, Angew. Chem., Int. Ed., 2011, 50, 8753 CrossRef CAS PubMed;
(c) Z. Xiang and D. Cao, Macromol. Rapid Commun., 2012, 33, 1184 CrossRef CAS PubMed;
(d) J. L. Novotney and W. R. Dichtel, ACS Macro Lett., 2013, 2, 423 CrossRef CAS.
-
(a) L. Chen, Y. Honsho, S. Seki and D. Jiang, J. Am. Chem. Soc., 2010, 132, 6742 CrossRef CAS PubMed;
(b) Y. Xu, L. Chen, Z. Cuo, A. Nagai and D. Jiang, J. Am. Chem. Soc., 2011, 133, 17622 CrossRef CAS PubMed;
(c) J. Jiang, A. Trewin, D. Adams and A. I. Cooper, Chem. Sci., 2011, 2, 1777 RSC;
(d) Q. Chen, J. Wang, F. Yang, D. Zhou, N. Bian, X. Zhang, C. Yan and B. Han, J. Mater. Chem., 2011, 21, 13554 RSC;
(e) C. Gu, Y. Chen, Z. Zhang, S. Xue, S. Sun, K. Zhang, C. Zhong, H. Zhang, Y. Pan, Y. Lv, Y. Yang, F. Li, S. Zhang, F. Huang and Y. Ma, Adv. Mater., 2013, 25, 3443 CrossRef CAS PubMed;
(f) Y. Wei, W. Chen, X. Zhao, S. Ding, S. Han and L. Chen, Polym. Chem., 2016, 7, 3983 RSC.
-
(a) Z. Xie, C. Wang, K. E. deKrafft and W. Lin, J. Am. Chem. Soc., 2011, 133, 2056 CrossRef CAS PubMed;
(b) K. Zhang, D. Kopetzki, P. Seeberger, M. Antonietti and F. Vilela, Angew. Chem., Int. Ed., 2013, 52, 1432 CrossRef CAS PubMed;
(c) R. Li, Z. Wang, L. Wang, B. Ma, S. Ghasimi, H. Lu, K. Landfester and K. Zhang, ACS Catal., 2016, 6, 1113 CrossRef CAS;
(d) C. Yang, B. Ma, L. Zhang, S. Lin, S. Ghasimi, K. Landfester, K. Zhang and X. Wang, Angew. Chem., Int. Ed., 2016, 55, 9202 CrossRef CAS PubMed;
(e) R. S. Sprick, J.-X. Jiang, B. Bonillo, S. Ren, T. Ratvijitvech, P. Guiglion, M. A. Zwijnenburg, D. J. Adams and A. I. Cooper, J. Am. Chem. Soc., 2015, 137, 3265 CrossRef CAS PubMed.
-
(a) F. Vilela, K. Zhang and M. Antonietti, Energy Environ. Sci., 2012, 5, 7819 RSC;
(b) Z. Xiang and D. Cao, J. Mater. Chem. A, 2013, 1, 2691 RSC;
(c) J.-K. Sun and Q. Xu, Energy Environ. Sci., 2014, 7, 2071 RSC;
(d) Z.-S. Wu, L. Chen, J. Liu, K. Parvez, H. Liang, J. Shu, H. Sachdev, R. Graf, X. Feng and K. Muellen, Adv. Mater., 2014, 26, 1450 CrossRef CAS PubMed;
(e) F. Xu, X. Chen, Z. Tang, D. Wu, R. Fu and D. Jiang, Chem. Commun., 2014, 50, 4788 RSC;
(f) H. Liao, H. Ding, B. Li, X. Ai and C. Wang, J. Mater. Chem. A, 2014, 2, 8854 RSC.
-
(a) A. G. Slater and A. I. Cooper, Science, 2015, 348, 988 CrossRef CAS PubMed;
(b) H. Ren and G. Zhu, Acta Chim. Sin., 2015, 73, 587 CrossRef CAS;
(c) Q. Liu, Z. Tang, M. Wu and Z. Zhou, Polym. Int., 2014, 63, 381 CrossRef CAS;
(d) B. Bonillo, R. S. Sprick and A. I. Cooper, Chem. Mater., 2016, 28, 3469 CrossRef CAS.
-
(a) G. Cheng, T. Hasell, A. Trewin, D. J. Adams and A. I. Cooper, Angew. Chem., Int. Ed., 2012, 51, 12727 CrossRef CAS PubMed;
(b) P. Zhang, Z. Weng, J. Guo and C. Wang, Chem. Mater., 2011, 23, 5243 CrossRef CAS;
(c) S. Deng, J. Zhi, X. Zhang, Q. Wu, Y. Ding and A. Hu, Angew. Chem., Int. Ed., 2014, 53, 14144 CrossRef CAS PubMed.
-
(a) J.-X. Jiang, F. Su, H. Niu, C. D. Wood, N. L. Campbell, Y. Z. Khimyak and A. I. Cooper, Chem. Commun., 2008, 486 RSC;
(b) K. Wu, J. Guo and C. Wang, Chem. Mater., 2014, 26, 6241 CrossRef CAS.
-
(a) A. S. Hay, J. Org. Chem., 1962, 27, 3320 CrossRef CAS;
(b) R. Du, N. Zhang, H. Xu, N. Mao, W. Duan, J. Wang, Q. Zhao, Z. Liu and J. Zhang, Adv. Mater., 2014, 26, 8053 CrossRef CAS PubMed.
- P.-Z. Li, X.-J. Wang, S. Y. Tan, C. Y. Ang, H. Chen, J. Liu, R. Zou and Y. Zhao, Angew. Chem., Int. Ed., 2015, 54, 12748 CrossRef CAS PubMed.
- D. Sahu, C.-H. Tsai, H.-Y. Wei, K.-C. Ho, F.-C. Chang and C.-W. Chu, J. Mater. Chem., 2012, 22, 7945 RSC.
- P. Pandey, O. K. Farha, A. M. Spokoyny, C. A. Mirkin, M. G. Kanatzidis, J. T. Hupp and S. T. Nguyen, J. Mater. Chem., 2011, 21, 1700 RSC.
- S. Yuan, S. Kirklin, B. Dorney, D. Liu and L. Yu, Macromolecules, 2009, 42, 1554 CrossRef CAS.
- D.-W. Wang, F. Li, M. Liu, G. Q. Lu and H.-M. Cheng, Angew. Chem., Int. Ed., 2008, 47, 373 CrossRef CAS PubMed.
- Y. Zeng, R. Zou and Y. Zhao, Adv. Mater., 2016, 28, 2855 CrossRef CAS PubMed.
- A. Li, L. Wang, X. Wang, K. Han and W. Deng, Angew. Chem., Int. Ed., 2010, 49, 3330 CrossRef CAS PubMed.
Footnotes |
† Electronic supplementary information (ESI) available: Experimental section and characterization results. See DOI: 10.1039/c6qm00190d |
‡ These authors contributed equally to this work. |
|
This journal is © the Partner Organisations 2017 |
Click here to see how this site uses Cookies. View our privacy policy here.