DOI:
10.1039/C6QM00270F
(Research Article)
Mater. Chem. Front., 2017,
1, 937-946
Combination therapy of pDNA and siRNA by versatile carriers composed of poly(L-serine) modified polyethylenimines
Received
20th October 2016
, Accepted 30th November 2016
First published on 12th December 2016
Abstract
Multi-gene-based approaches for tumor therapy have yet to be developed. Accordingly, we explored a combination treatment strategy by loading two kinds of therapeutic reagents simultaneously: pDNA and siRNA. Polyethylenimine–poly(L-serine) (PEI–PSer) obtained via ring-opening polymerization of L-serine N-carboxyanhydride initiated by polyethylenimines served as a potent cationic gene carrier. Proton nuclear magnetic resonance spectroscopy and gel permeation chromatography confirmed the successful preparation of PEI–PSer copolymers. The morphologies and effective binding capacities of PEI–PSer and genes were evaluated through gel retardation, scanning electron microscopy, and particle size and zeta potential assays. PEI–PSer displayed pDNA transfection and siRNA knockdown efficacy without affecting cell viability. The high gene transfer efficacy was mainly due to the excellent intracellular trafficking ability of PEI–PSer, as confirmed by confocal laser scanning microscopy and flow cytometry. Cell apoptosis assay further confirmed the combination antitumor effect aroused by the co-delivery of pKH-rev-casp-3 and siBcl2 by PEI–PSer. This study introduced a new carrier that delivers pDNA and siRNA simultaneously, thereby providing a potential strategy for tumor treatment.
Introduction
Malignant tumors have become the major threat to human health. A single treatment strategy cannot effectively treat tumors because multiple factors affect the occurrence, development, and metastasis of tumors.1,2 Therefore, combination treatments are effective for treating tumors.3 Combined strategies, such as surgery and radiotherapy, radiotherapy and chemotherapy, chemotherapy and gene therapy, and chemotherapy and photodynamic therapy, are currently being developed to improve the comprehensive performance of tumor therapies.4–8 However, the practical applications of such combined strategies are limited by (1) multidrug resistance secondary to long-term chemotherapy, (2) poor adaptability and increased suffering in patients secondary to side effects, and (3) high costs.
To solve these problems, gene therapy has shown tremendous potential in clinical applications for treating tumors in recent years.9–13 Moreover, the gradual accumulation of multi-gene mutations is likely to cause tumors.14 Multi-gene-based approaches greatly widen the clinical applications of nanoparticle-based gene therapies for precise tumor treatment. In general, therapeutic nucleic acids can be divided into pDNA, antisense ODNs, mRNA, small interfering RNA (siRNA), and microRNA. The mechanisms of nucleic acid therapies depend on the types of genes: pDNA must be transported to the nucleus, whereas other nucleic acids require an early release from the endosome and work in the cytoplasm.15 However, genes themselves are difficult to apply directly because of their rapid degradation in plasma and poor intracellular uptake.16–18
Therefore, gene carriers are important in successful gene therapies.19,20 Viral vectors have been developed to deliver therapeutic genes into the lesion sites,21 but their broad application is restricted by safety concerns.22 Therefore, various non-viral vehicles, such as lipoplex particles,23 polyplex particles,24 self-assembling particles,6 and inorganic nanoparticles,25 have been developed extensively as safe and reliable gene carriers.19 Cationic polyplex particles are popular gene carriers because of their superior solubility and ability to condense DNA/RNA via electrostatic interaction.19,26 Gene delivery systems with cationic components show outstanding performance because they can attach to the anionic surface of the plasma membrane, thereby allowing uninhibited entry into the vacuolar compartments of tumor cells.17 However, most carriers are efficient for just one gene (DNA or RNA), whereas a few can achieve their potential abilities of loading multi-nucleic acids of different natures simultaneously.15 Furthermore, therapeutic genes require appropriate delivery systems depending on their properties, including size, structure, and chemistry. Therefore, developing safe and effective multi-gene-based gene delivery systems is urgently needed.
Polyethylenimine (PEI) is the gold standard in cationic carriers for the in vitro delivery of pDNA or siRNA.27,28 However, the cytotoxicity aroused by the highly positively charged density of PEI limits its further application in vivo. To realize multi-gene-based approaches and improve PEI performance, we explored potent cationic gene carriers (PEI–PSer) obtained via ring-opening polymerization of L-serine N-carboxyanhydride (Ser(OBz)–NCA) initiated by polyethylenimines for the combination treatment strategy by loading two kinds of therapeutic reagents simultaneously: pDNA and siRNA (Scheme 1). The physiochemical properties, transfection efficacy, and intracellular uptake of PEI–PSer for both pDNA and siRNA were also investigated. Furthermore, the combination effect of the pKH3-rev-casp-329 and siBcl230 double-gene-based strategy by PEI–PSer was explored.
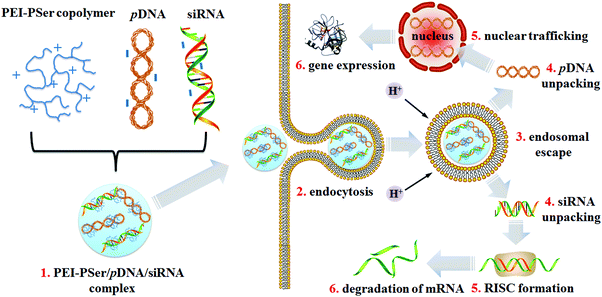 |
| Scheme 1 The schematic diagram of complex formation, endocytosis and intracellular trafficking. | |
Results and discussion
Synthesis and characterization of PEI–PSer copolymers
PEI–Psers were synthesized via one-step ring-opening polymerization of Ser(OBz)–NCA monomers using branched PEI-25k as the initiator. Then, PEI–PSer were obtained by removing the protected benzyl groups using HBr. The synthesis of PEI–PSer is shown in Fig. 1. The 1H NMR spectrum of PEI–PSer was analyzed. The peaks at 2.5–3.5 and 3.7–4.2 ppm were assigned to PEI and Pser, respectively (Fig. 2A). The gel permeation chromatography (GPC) profile (Fig. 2B) of PEI–PSer exhibited a unimodal peak, implying successful PEI–PSer synthesis.
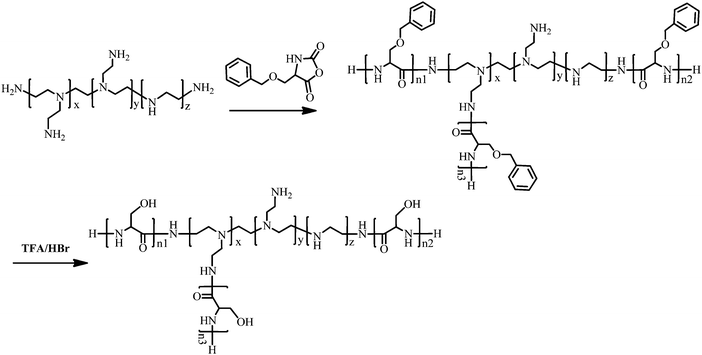 |
| Fig. 1 Synthesis route to poly(L-serine) modified polyethylenimines (PEI–PSer). | |
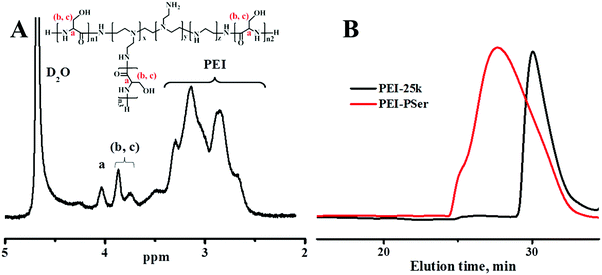 |
| Fig. 2 (A) 1H NMR spectra of PEI–PSer2 in D2O and (B) GPC of PEI-25k and PEI–PSer2. | |
The number-average molecular weight (Mn) gradually increased as the molar ratio of PSer/PEI-25k increased. Moreover, PEI–PSer exhibited a larger number-average molecular weight (Mn) than the theoretical value. The possible reasons were (1) PEI–PSer contained several polyserine groups that enhanced the interaction between polymers to form hydrogen bonds, thereby forming large particles; and (2) the standard samples used in this work were linear PEGs, which have different chemical structures compared to PEI and PEI–PSers (Table 1).
Table 1 Molecular weights of PEI-25k and PEI–PSer
Polymer |
Theoretical mol ratio PEI/PSer |
Resultant mol ratio by 1H NMR PEI/PSer |
Theoretical Mn (kDa) |
Resultant Mn (kDa) by 1H NMR |
GPC result |
M
n (kDa) |
M
w (kDa) |
Polydispersity (Mw/Mn) |
PEI-25k |
— |
— |
10 |
— |
11.9 |
14.3 |
1.19 |
PEI–PSer1 |
1/30 |
1/36 |
12.6 |
13.1 |
32.1 |
60.5 |
1.88 |
PEI–PSer2 |
1/60 |
1/63 |
15.2 |
15.5 |
39.4 |
60.3 |
1.52 |
PEI–PSer3 |
1/90 |
1/86 |
17.8 |
17.6 |
40.3 |
62.3 |
1.54 |
Characterization of PEI–PSer/gene complexes
To identify the formation of PEI–PSer/gene complexes, the electrostatic interaction between positively charged PEI–PSer and negatively charged pDNA (or siRNA) was performed using agarose gel electrophoresis. As shown in Fig. 3, the movement of genes was retarded as the amount of cationic polymers increased mainly because of the neutralization of negatively charged genes. The completely retardant ratios of the PEI–PSer2/pDNA and PEI–PSer2/siRNA complexes were achieved at weight ratios of 0.4
:
1 and 0.5
:
1, respectively. For the commercial PEI-25k, the completely retardant ratios were the same (0.3
:
1) and less than those of PEI–PSer2. The higher retarded ratio of PEI–PSer/gene, compared with that of PEI-25k/gene, was mainly due to its lower positive-charge density. In this study, PEI–PSer was synthesized via one-step ring-opening polymerization of Ser(OBz)–NCA monomers with PEI-25k as the initiator. Moreover, the biocompatible polyserine moieties were introduced to shield the positive charge on the surface of PEI-25k. Therefore, the positive-charge density of PEI–PSer was lower than that of PEI-25k, and this result was further verified by zeta potential assay (Fig. 5).
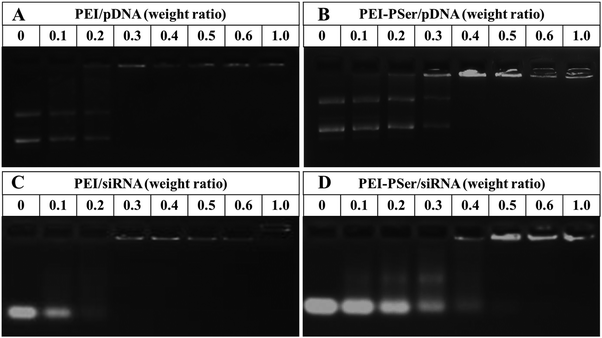 |
| Fig. 3 Gel retardation assay of carriers/gene complexes with various weight ratios of the carriers conjugated to pDNA or siRNA. (A) PEI-25k/pDNA, (B) PEI–PSer2/pDNA, (C) PEI-25k/siRNA, and (D) PEI–PSer2/siRNA. | |
Suitability of size is essential for efficient endocytosis and cationic carrier transfection.24 In the present study, the particle sizes of the complexes formed by PEI-25k/pDNA/siRNA and PEI–PSer/pDNA/siRNA were measured using a zeta potential/BI-90 Plus particle size analyzer on the basis of their transfection weight ratios. As shown in Fig. 4A, PEI–PSer could condense pDNA and siRNA effectively to form nanoparticles 130.5–195.6 nm in size (PDI = 0.094–0.169), showing that the PEI–PSer copolymer possessed a property similar to that of PEI-25k (PDI = 0.103–0.184) for compacting nucleic acids into nanoparticles. Fig. 4B and C show the transmission electron microscopy (TEM) images of the PEI-25k/pDNA/siRNA and PEI–PSer2/pDNA/siRNA complexes, respectively. The sizes of the PEI-25k/pDNA/siRNA and PEI–PSer/pDNA/siRNA complexes were smaller than those obtained from the previous results mainly because of the shrinkage of the samples caused by the preparation of drying samples. The results could further verify the formation of PEI–PSer2/pDNA/siRNA nanoparticles suited for gene delivery.
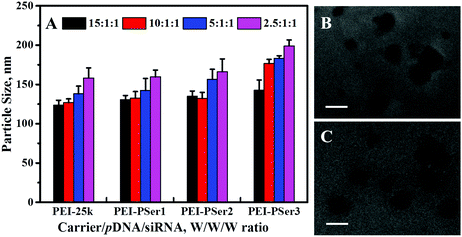 |
| Fig. 4 Particle size of PEI-25k/pDNA/siRNA and PEI–PSer/pDNA/siRNA complexes at their various weight ratios (A) and the TEM images of the optimal transfection ratios of PEI-25k/pDNA/siRNA (B) and PEI–PSer2/pDNA/siRNA (C). All complexing ratios are expressed as weight ratios. Data are shown as mean ± SD (n = 4), Scale bar = 100 μm. | |
The membranes of cancer cells are negatively charged; therefore, positively charged nanoparticles can easily approach and contact cellular membranes and be smoothly absorbed. Fig. 5 shows the zeta potentials of the PEI-25k/pDNA/siRNA and PEI–PSer/pDNA/siRNA complexes at various weight ratios. The zeta potentials of the complexes gradually improved as the carrier-to-gene ratio increased. Moreover, the zeta potentials of the nanoparticles formed by PEI–PSer/pDNA/siRNA were lower those that of PEI-25k/pDNA/siRNA in the same weight ratio. This result could be attributed to the reduced charge density of PEI–PSer secondary to the introduction of polyserine segments.
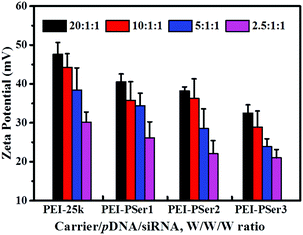 |
| Fig. 5 Zeta potentials of PEI–PSer2/pDNA/siRNA complexes in comparison with PEI-25k/pDNA/siRNA at various transfection weight ratios. The data are expressed as mean ± SD (n = 3). | |
Cytotoxicity assay of PEI–PSer/pDNA/siRNA complexes
The clinical application of cationic nanoparticle-mediated gene therapy is limited by the cytotoxicity resulting from the reaction between cationic components and proteoglycans on the cell membrane; this cytotoxicity causes cytomembrane destabilization and meronecrobiosis.17 The cytotoxicities of PEI-25k/pDNA/siRNA and PEI–PSer/pDNA/siRNA were assessed using MTT assay at various concentrations in HeLa and 293T-GFP cells. As shown in Fig. 6, the complexes formed by PEI–PSer conjugates and nucleic acids (pDNA and siRNA) showed significantly higher cell viability and reduced toxicity compared with those formed by PEI-25k in all test cell lines. Moreover, the cytotoxicity of the PEI–PSer copolymers slightly decreased as the degree of substitution increased. In general, the cytotoxicity of PEI-derived gene carriers is correlated with their surface charge density.31 The results further suggest that the novel delivery systems showed good biocompatibility, which could be attributed to the decreased concentration of primary amine groups after introducing polyserine segments.
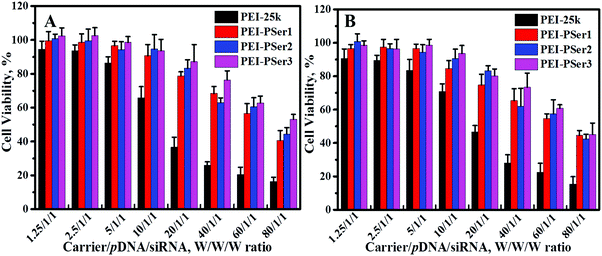 |
| Fig. 6 Viability of HeLa (A) and 293T-GFP (B) cells exposed to various ratios of PEI-25k/pDNA/siRNA and PEI–PSer/pDNA/siRNA. The data represent mean ± SD (n = 4). | |
In vitro transfection activity and gene silencing effect
The transfection efficacies of PEI–PSer and PEI-25k were evaluated using luciferase assay in 293T-GFP cell lines with plasmid DNA (pGL3-control) as the reporter gene. As shown in Fig. 7, the PEI–PSer copolymers exhibited higher transfection efficacies in both cell lines compared with PEI-25k in the optimal weight ratios of carrier/pDNA/siRNA. Notably, the carrier/pDNA/siRNA weight ratio achieved by the optimal transfection efficacy increased gradually for PEI–PSer1, PEI–PSer2, and PEI–PSer3. This phenomenon was mainly due to the increased polyserine content and consequent decreased charge densities in the PEI–PSer copolymers. Therefore, the amount of PEI–PSer3 must be increased to combine with pDNA efficiently and achieve competent gene transfection. Among the PEI–PSer conjugates, PEI–PSer2 demonstrated the most efficient gene transfection, which was 4.8 and 3.8 times higher than those of PEI in HeLa-GFP and 293T-GFP cells, respectively.
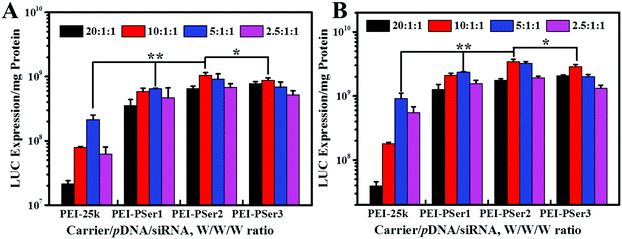 |
| Fig. 7
In vitro gene transfection efficiency of PEI–PSer/pGL3-control/siGFP and PEI-25k/pGL3-control/siGFP complexes for 48 h in HeLa-GFP (A) and 293T-GFP (B) cells. The final concentrations of pGL3-control and siGFP were 1 μg mL−1. Data represent mean ± SD with three replicates. **P < 0.001, *P < 0.01. | |
RNA-mediated targeted gene silencing technology is a promising method to treat numerous gene-related diseases. A safe and effective delivery system is key for RNA-based therapies. As reported, carriers that are efficient for plasmid DNA are usually ineffective for siRNA delivery.15 In this regard, the siRNA delivery efficacy of PEI–PSer copolymers was assessed in a green fluorescent protein (GFP) that stably expresses 293T cells using siRNA against the GFP. Gene silencing activities were evaluated by treating the cells with either GFP siRNA or control Rev siRNA, followed by comparing them with the green fluorescence of untreated cells. Knockdown effects were positive when fluorescence intensity decreased with GFP siRNA but not with the control siRNA. As shown in Fig. 8, the PEI–PSer copolymers exhibited the highest fluorescence knockdown efficacy when formulated with GFP siRNA in 293T-GFP cells. In the commercial PEI-25k, a slight gene knockdown effect was achieved. No toxicity and silencing effect were observed when only two types of siRNAs were used. Furthermore, the intensity of the fluorescent photographs was quantified and analyzed using NIH ImageJ. The fluorescence intensity of the PEI–PSer/pGL3-control/siGFP-treated group decreased significantly compared with that of the PEI-25k/pGL3-control/siGFP group after 48 h. Moreover, the ratios of fluorescence intensity decreased to approximately 69.9% and 37.7%, respectively. However, the fluorescence intensity of the control groups was not significantly reduced. In consideration of the previous gene transfection results, the PEI–PSer2 copolymer could be a versatile carrier for nucleic acid (pDNA and/or siRNA) delivery, thereby providing a potential strategy for treating gene-related diseases.
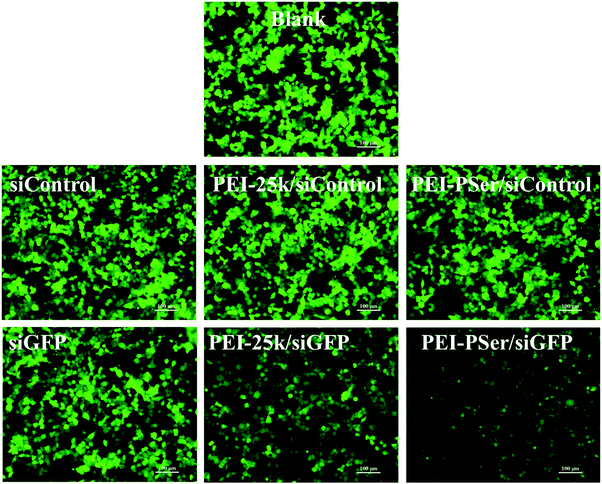 |
| Fig. 8 Gene silencing of PEI–PSer2/pGL3-control/siGFP and PEI-25k/pGL3-control/siGFP complexes in 293T-GFP cells. Scale bar = 100 μm. | |
Intracellular uptake of pDNA and siRNA complexes
Positively charged nanoparticles could easily adhere to the negatively charged membranes of tumor cells and could be effectively internalized.31 To investigate the influence of polyserine segment modification in PEI-25k on transfection efficacy, the intracellular uptake of fluorescent-labeled pDNA (Cy5-DNA) and siRNA (FAM-siRNA) formulations into HeLa cells were evaluated using confocal laser scanning microscopy (CLSM) (Fig. 9) and flow cytometry (FCM) (Fig. 10) assays with optimal carrier/pDNA/siRNA ratios.
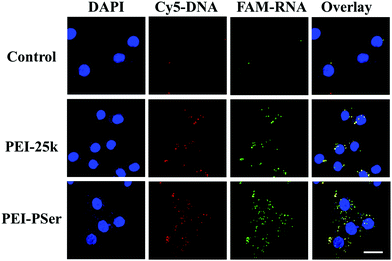 |
| Fig. 9 CLSM images of HeLa cells incubated with naked pDNA/siRNA, PEI-25k/pDNA/siRNA and PEI–PSer2/pDNA/siRNA complexes. DAPI, cell nucleus (blue); CY5-DNA (red); FAM-RNA (green). Scale bars = 20 μm. | |
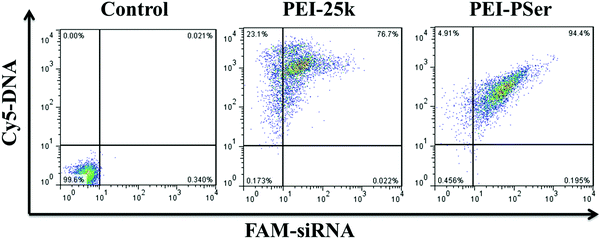 |
| Fig. 10 Cellular uptake of PEI-25k/pDNA/siRNA and PEI–PSer2/pDNA/siRNA in their optimal transfection ratios in HeLa cells. | |
As shown in Fig. 9, high levels of red and green fluorescence were observed in the cells of all tested carriers. The results revealed highly efficient pDNA and siRNA co-intracellular trafficking, whereas mild fluorescence was observed in the cells with naked pDNA and siRNA, further validating that free genes cannot easily enter cells and need proper gene delivery systems. Although strong fluorescent signals were observed with PEI-25k and PEI–PSer2, more intense red and green fluorescence signals were observed in the PEI–PSer2 group, suggesting that the PEI–PSer2/pDNA/siRNA complex achieved a more efficient cell endocytosis. The enhanced intracellular uptake phenomenon was mainly attributed to the introduction of polyserine groups.
The internalization efficacy was further monitored using FCM in HeLa cells. The cells were cultured with the PEI-25k/pDNA/siRNA and PEI–PSer2/pDNA/siRNA complexes for 4 h, and the intracellular gene uptake was analyzed. As shown in Fig. 10, the horizontal and vertical axes represent Cy5-DNA and FAM-siRNA, respectively. The data for the right upper quadrant indicate the co-location percentages of DNA and siRNA in all the detected cells. The internalization efficacy of PEI–PSer (94.4%) was significantly higher than that of PEI-25k (76.7%) in HeLa cells. Moreover, the enhanced cellular uptake capacity of PEI–PSer is in agreement with our previous CLSM observation. Overall, the results suggest that the PEI–PSer copolymers could more efficiently mediate the entry of DNA and siRNA into tumor cells in the serum-containing medium compared with commercial PEI-25k.
Collaborative treatment of pDNA and siRNA by PEI–PSer copolymers
To confirm the collaborative treatment of pDNA and siRNA induced by the PEI–PSer copolymers, cell apoptosis of the tumor cells was evaluated after treatment with the PEI-25k/pDNA/siRNA and PEI–PSer/pDNA/siRNA complexes for 48 h. The Rev-casp-3 gene, which regulates programmed cell death, was selected as a therapeutic gene to induce tumor apoptosis.29 Moreover, Bcl2 plays an important role in the anti-apoptotic proteins and is related to the mitochondrial pathway of cell apoptosis.30 The co-delivery of pKH-rev-casp-3 and Bcl2 siRNA by the same carrier was expected to achieve combination effects in tumor therapy. As shown in Fig. 11, the control group demonstrated a relatively low apoptotic rate (1.0%). The cells treated with PEI–PSer/pKH-rev-casp-3/siBcl2 had a higher apoptotic rate (50.9%) than those treated with pKH-rev-casp-3/siBcl2 (9.9%), PEI–PSer2/pKH-rev-casp-3 (15.9%), and PEI–PSer2/siBcl2 (44.6%) (data not shown). PEI-25k, as a positive control in this study, was used as the gold standard in cationic carriers for in vitro gene delivery.27,28 The PEI-25k/pKH-rev-casp-3/siBcl2 group also showed an obvious cell apoptosis ratio (44.3%). However, the cytotoxicity aroused by the highly positively charged density of PEI limits its further application. Therefore, PEI–PSer was explored in this study. One the one hand, PEI–PSer showed lower cytotoxicity than PEI-25k (Fig. 6). On the other hand, PEI–PSer induced a stronger capacity of gene transfection (Fig. 7) and cell apoptosis (Fig. 11) than PEI-25k.
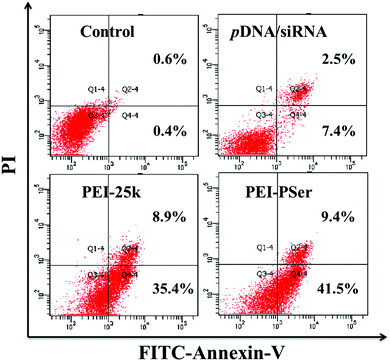 |
| Fig. 11 Cell apoptosis of HeLa cells treated with pKH-rev-casp-3/siBcl2, PEI-25k/pKH-rev-casp-3/siBcl2 and PEI–PSer2/pKH-rev-casp-3/siBcl2 for 48 h, respectively. | |
Conclusion
We developed PEI–PSer copolymers into a novel and versatile pDNA and siRNA co-delivery system. PEI–PSer were synthesized via ring-opening polymerization of L-serine N-carboxyanhydride initiated by polyethylenimines. The structures of the PEI–PSer copolymers were confirmed using 1H NMR and GPC tests. The results of gel retardation, TEM, particle size, and zeta potential measurements revealed that the PEI–PSer copolymers showed effective binding capacities with genes. Furthermore, the PEI–PSer displayed unanticipated gene transfection efficacy and knockdown effects secondary to complexing with model pDNA and siRNA without significantly affecting cell viability. The excellent gene delivery was mainly due to the excellent intracellular trafficking ability acquired from confocal imaging and FCM. Cell apoptosis assay further confirmed the combination antitumor effect aroused by the co-delivery of therapeutic pDNA and siRNA using the PEI–PSer copolymers. Overall, the data demonstrated a new vehicle for simultaneously co-delivering pDNA and siRNA, providing a potential strategy for tumor treatment. Moreover, in vivo studies are underway in our laboratory. Further investigation of the antitumor therapy of multi-gene-based approaches in combination therapy by systemic administration is needed. Moreover, introducing targeted moieties will likely improve the selectivity of gene delivery systems for antitumor therapies via systematic administration in vivo.
Experimental section
Materials and methods
Serine and branched PEI with a weight-average molecular weight of 25 kDa (PEI-25k) were purchased from Aldrich (St Louis, MO, USA). NCA of serine (Ser(OBz)–NCA) was purchased from GL Biochem Ltd. (Shanghai, China). Chloroform was refluxed with calcium hydride (CaH2) and distilled under nitrogen prior to use. 3-(4,5-Dimethylthiazol-2-yl)-2,5-diphenyltetrazolium bromide (MTT) was purchased from Amresco (Solon, Ohio, USA). The luciferase plasmid (pGL3-control), cell lysates and the luciferase reporter gene assay kit were purchased from Promega (Wisconsin, USA). The Rev-casp-3 gene was subcloned into a pKH3 vector and designed according to our previously reported method.29 Two Bcl2 siRNA duplexes (Bcl2-1 and Bcl2-2) were purchased from Genepharma (Suzhou, China) with the sequences as follows: Bcl2-1 siRNA 5′-UGU GGA UGA CUG AGU ACC UGAdTdT-3′ (sense), 5′-UCA GGU ACU CAG UCA UCC ACAdTdT-3′ (antisense); Bcl2-2 siRNA 5′-GUA CAU CCA UUA UAA GCU GUCdTdT-3′ (sense), 5′-GAU AGC UUA UAA UGG AUG UACdTdT-3′ (antisense). The mixture of Bcl2-1 siRNA and Bcl2-2 siRNA was in an equal molar ratio and named as Bcl2 siRNA. Cy5-labeled DNA was purchased from RiboBio (Guangzhou, China). All other chemicals were purchased from Sinopharm Chemical reagent Co. Ltd (China) without further treatment.
Synthesis of PEI–PSer and structural characterization
PEI–PSer were prepared via one-step ring opening polymerization (Fig. 1). Branched PEI-25k was mixed with Ser-NCA in different molar ratios in anhydrous chloroform, and the molar ratios of PEI
:
Ser(OBz) were 1
:
30, 1
:
60, and 1
:
90. The mixtures were stirred at 30 °C for 72 h. The solutions were then concentrated and precipitated in cold diethyl ether. After filtration, the PEI–PSer copolymers were obtained and vacuum dried for 24 h at room temperature. PEI–PSer(OBz)s were dissolved in TFA and the protecting group was removed using HBr. The solution was concentrated and precipitated in cold diethyl ether. After filtration, PEI–PSers were obtained and vacuum dried for 24 h at room temperature. The obtained product was dissolved in distilled water and then dialyzed (molecular weight cutoff 7000) against water (500 mL, 4 times over 48 h). The light yellow powder was harvested via lyophilization.
1H NMR spectra of PEI–PSer were recorded on a Bruker AV400 NMR spectrometer (Bruker, Ettlingen, Germany) at room temperature using denatured water as the solvent. The molecular weights (Mw and Mn) and distributions (polydispersity index = Mw/Mn) of the PEI–PSer copolymers were determined using a GPC system (Waters Corp, Milford, MA, USA), a Waters 515 pump and a series of linear Styragel columns. NaAc/HAc buffer (0.3 M, pH 4.4) was used as the eluent with a flow rate of 1.0 mL min−1 at 40 °C.
Particle size, zeta potential, and TEM assays
PEI–PSer/pDNA/siRNA complexes were prepared at the weight ratio of 5
:
1
:
1 in distilled water, and both of the final concentrations of pDNA and siRNA were 10 μg mL−1. After 30 min of incubation at room temperature, the particle size and zeta potential measurements of the complex solutions were performed using a zeta potential/BI-90 Plus particle size analyzer at room temperature. The morphology and sizes were examined using TEM. The TEM samples were prepared as follows: 1 drop of carrier/pDNA/siRNA complex solution (the carrier concentration was 0.05 mg mL−1) was deposited onto a 200-mesh copper grid coated with carbon and then dried at room temperature. The TEM figures were obtained using a JEOL JEM-1011 electron microscope operating at an acceleration voltage of 100 kV.
Gel retardation assay
The pDNA binding capacity of the PEI–PSer copolymer was evaluated by gel retardation assay. The complexes were prepared at various weight ratios of 0, 0.1, 0.2, 0.3, 0.4, 0.5, 0.6 and 1.0. The loading amount of pDNA was 0.5 μg per well. After incubation for 20 min at room temperature, 2 μL of 6× loading buffer was added into each well. Electrophoresis was carried out on 1% (W/V) agarose gel at 100 V for 40 min in TAE buffer solution (40 mmol L−1 Tris-HCl, 1 vol% acetic acid and 1 mmol L−1 EDTA). After staining with ethidium bromide, the retardation of pDNA was visualized with a UV lamp using a UVP EC3 bioimaging system (UVP, Inc., Upland, CA, USA). The protocol of siRNA binding ability was referred to that of pDNA, except that the electrophoresis was carried out on 2% agarose gel at 80 V for 20 min and all the reagents were pre-treated with diethyl phosphorocyanidate (DEPC).
Cell culture and MTT assays
For pDNA and siRNA delivery experiments, human cervical carcinoma cells (HeLa cells) and human embryonic kidney (293T-GFP cells) stably transfected with green fluorescent protein gene were carried out in this study. All cells were cultured in Dulbecco's modified Eagle's medium (DMEM) high glucose, supplemented with 10 vol% of heat-inactivated FBS, 100 units mL−1 penicillin and 63 mg mL−1 streptomycin in a 5% CO2 incubator at 37 °C under 95% humidity.
The cytotoxicity of PEI–PSer/pDNA/siRNA complexes was evaluated by MTT assay.32,33 Briefly, the cells were seeded in 96-well plates at a density of 1.0 × 104 cells per well and incubated at 37 °C in 5% CO2 incubator for 24 h before treating with various complexes solutions at the different weight ratios range from 1.25
:
1
:
1 to 80
:
1
:
1. PEI-25k was utilized as the control and both of the final concentrations of pDNA and siRNA were 1 μg mL−1. After incubation for 48 h, 20 μL of MTT stock solution (5 mg mL−1 in PBS) was added. The culture medium was carefully removed after 4 h and 150 μL DMSO was added to dissolve the MTT formazan crystals. The absorbance (492 nm) was analyzed using an ELISA microplate reader (Bio-Rad). The cell viability was normalized to that of the cells cultured only in the DMEM medium. Each experiment was performed in quadruplicate on at least three occasions.
Gene transfection and gene silencing experiment
Gene transfection and gene silencing experiments were performed using HeLa-GFP and 293T-GFP cells. In brief, the cells were seeded in 96-well plates at 1.0 × 104 cells per well and then incubated for 24 h until the cells were 80% confluent. The medium was replaced with fresh DMEM containing PEI-25k/pGL3-control/siGFP or PEI–PSer/pGL3-control/siGFP complexes at their various weight ratios from 2.5
:
1
:
1 to 20
:
1
:
1. The final concentration of pGL3-control was 1 μg mL−1. For gene transfection analysis, the cells were incubated for another 48 h and then lysed thoroughly using cell lysis buffer (Promega, 50 μL per well). The luciferase expression was measured for 10 s using a luciferase assay kit (GloMax 20/20, Promega), and the total protein content of the cell lysate was determined by a BCA Protein Assay Kit (Thermo, Rockford, USA). The luciferase activity was expressed as luciferase expression per mg protein. For GFP gene silencing, the cells were incubated with the carrier/pGL3-control/siGFP complex for 48 h. Furthermore, the fluorescent photographs were obtained using a microscope in a dark environment.
FCM and CLSM assay for intracellular uptake
To verify intracellular translocation, carrier/pDNA/siRNA complexes were evaluated in HeLa cells using CY5-labeled pDNA and FAM-labeled siRNA via CLSM and FCM.
For CLSM, HeLa cells (2.5 × 105 cells per well) were seeded in a 6-well plate with a coverslip in each well and then incubated for 24 h. The medium was replaced with complete DMEM medium containing PEI-25k/pDNA/siRNA or PEI–PSer/pDNA/siRNA. The final concentrations of CY5-DNA and FAM-RNA were 0.5 μg mL−1 in all experimental groups. The cells were incubated with the complexes for 4 h and then fixed in paraformaldehyde (PFA, 4% in PBS) for 10 min at room temperature. After immobilization, the coverslips were washed thrice with PBS, and then the nuclei were stained with DAPI (0.5 μg mL−1) for 5 min. Finally, the coverslips were washed five times with PBS and enclosed with glycerol. The cellular uptakes of the complexes were visualized using CLSM (LSM 780, Carl Zeiss Inc., Jena, Germany). Then, the fluorescence intensity was quantified and analyzed with NIH ImageJ.
For FCM, HeLa cells were seeded in 6-well plates at 5.0 × 105 cells per well in 2 mL of DMEM medium and then cultured for 24 h. The medium was replaced with DMEM medium containing PEI-25k/pDNA/siRNA or PEI–PSer/pDNA. The final concentrations of CY5-DNA and FAM-RNA were 0.5 μg mL−1 in all experimental groups. The cells were incubated with the complexes for 4 h at 37 °C and then washed twice with PBS. The cells were detached with 0.25% trypsin, neutralized with DMEM, and then washed and resuspended with cold PBS. Finally, the cellular uptake efficacy was evaluated using a Guava easyCyte 6-2L Base System (Merck Millipore, USA).
Cell apoptosis assay
The combination gene therapy of pKH3-rev-casp-3 and siBcl2 by PEI–PSer was explored by a cell apoptosis study. Briefly, HeLa cells cultured in 24-well plates were treated with PEI-25k/pKH3-rev-casp-3/siBcl2 or PEI–PSer/pKH3-rev-casp-3/siBcl2 complexes for 48 h. The apoptotic cells were stained with Annexin V and Propidium Iodide (PI) using the Annexin V-FITC apoptosis detection kit according to the manufacturer's instructions (BD Biosciences, San Jose, USA) and the results were analyzed by flow cytometry.
Acknowledgements
This work was supported by the National Natural Science Foundation of China (Grant No. 51303173, 51233004, 51390484, 21474104, 51403205, 51473029, 51503200 and 51520105004), “Ten thousand talent program” outstanding young scholars, Jilin province science and technology development program (20160204032GX) and the Fundamental Research Funds for the Central Universities (2412016KJ042).
Notes and references
- W. Q. Chen, R. S. Zheng, P. D. Baade, S. W. Zhang, H. M. Zeng, F. Bray, A. Jemal, X. Q. Yu and J. He, Ca-Cancer J. Clin., 2016, 66, 115–132 CrossRef PubMed.
- J. Chen, H. Liang, L. Lin, Z. P. Guo, P. J. Sun, M. W. Chen, H. Y. Tian, M. X. Deng and X. S. Chen, ACS Appl. Mater. Interfaces, 2016, 8, 31558–31566 CAS.
- N. Cao, D. Cheng, S. Y. Zou, H. Ai, J. M. Gao and X. T. Shuai, Biomaterials, 2011, 32, 2222–2232 CrossRef CAS PubMed.
- N. Leroi, N. E. Sounni, E. Van Overmeire, S. Blacher, R. Maree, J. Van Ginderachter, F. Lallemand, E. Lenaerts, P. Coucke, A. Noel and P. Martinive, OncoTargets Ther., 2015, 6, 36825–36837 Search PubMed.
- N. R. Datta, S. G. Ordonez, U. S. Gaipl, M. M. Paulides, H. Crezee, J. Gellermann, D. Marder, E. Puric and S. Bodis, Cancer Treat. Rev., 2015, 41, 742–753 CrossRef CAS PubMed.
- C. Q. Mao, J. Z. Du, T. M. Sun, Y. D. Yao, P. Z. Zhang, E. W. Song and J. Wang, Biomaterials, 2011, 32, 3124–3133 CrossRef CAS PubMed.
- Z. X. Jiao, J. Chen, H. Y. Tian and X. S. Chen, Acta Polym. Sin., 2015, 127–132 CAS.
- C. B. He, D. M. Liu and W. B. Lin, ACS Nano, 2015, 9, 991–1003 CrossRef CAS PubMed.
- X. W. Guan, Z. P. Guo, L. Lin, J. Chen, H. Y. Tian and X. S. Chen, Nano Lett., 2016, 16, 6823–6831 CrossRef CAS PubMed.
- J. L. Xia, H. Y. Tian, J. Chen, Z. P. Guo, L. Lin, H. Y. Yang and Z. C. Feng, Chin. J. Polym. Sci., 2016, 34, 316–323 CrossRef CAS.
- R. Hu, C. B. Yang, Y. C. Wang, G. M. Lin, W. Qin, Q. L. Ouyang, W. C. Law, Q. T. Nguyen, H. S. Yoon, X. M. Wang, K. T. Yong and B. Z. Tang, Nano Res., 2015, 8, 1563–1576 CrossRef CAS.
- M. Ogris, S. Brunner, S. Schuller, R. Kircheis and E. Wagner, Gene Ther., 1999, 6, 595–605 CrossRef CAS PubMed.
- J. Chen, Z. Guo, H. Tian and X. Chen, Mol. Ther.–Methods Clin. Dev., 2016, 3, 16023 CrossRef PubMed.
- L. E. MacConaill, C. D. Campbell, S. M. Kehoe, A. J. Bass, C. Hatton, L. L. Niu, M. Davis, K. L. Yao, M. Hanna, C. Mondal, L. Luongo, C. M. Emery, A. C. Baker, J. Philips, D. J. Goff, M. Fiorentino, M. A. Rubin, K. Polyak, J. Chan, Y. X. Wang, J. A. Fletcher, S. Santagata, G. Corso, F. Roviello, R. Shivdasani, M. W. Kieran, K. L. Ligon, C. D. Stiles, W. C. Hahn, M. L. Meyerson and L. A. Garraway, PLoS One, 2009, 4, e7887 Search PubMed.
- C. Scholz and E. Wagner, J. Controlled Release, 2012, 161, 554–565 CrossRef CAS PubMed.
- D. Castanotto and J. J. Rossi, Nature, 2009, 457, 426–433 CrossRef CAS PubMed.
- J. Chen, X. Dong, T. S. Feng, L. Lin, Z. P. Guo, J. L. Xia, H. Y. Tian and X. S. Chen, Acta Biomater., 2015, 26, 45–53 CrossRef CAS PubMed.
- H. Y. Tian, Z. P. Guo, L. Lin, Z. X. Jiao, J. Chen, S. Q. Gao, X. J. Zhu and X. S. Chen, J. Controlled Release, 2014, 174, 117–125 CrossRef CAS PubMed.
- H. Y. Tian, J. Chen and X. S. Chen, Small, 2013, 9, 2034–2044 CrossRef CAS PubMed.
- Y. Y. Yang, H. Hu, X. Wang, F. Yang, H. Shen, F. J. Xu and D. C. Wu, ACS Appl. Mater. Interfaces, 2015, 7, 12238–12248 CAS.
- N. Clement and J. C. Grieger, Mol. Ther.–Methods Clin. Dev., 2016, 3, 16002 CrossRef PubMed.
- X. Guo and L. Huang, Acc. Chem. Res., 2012, 45, 971–979 CrossRef CAS PubMed.
- L. Miao, Y. H. Wang, C. M. Lin, Y. Xiong, N. H. Chen, L. Zhang, W. Y. Kim and L. Huang, J. Controlled Release, 2015, 217, 27–41 CrossRef CAS PubMed.
- J. Chen, Z. X. Jiao, L. Lin, Z. P. Guo, C. N. Xu, Y. H. Li, H. Y. Tian and X. S. Chen, Chin. J. Polym. Sci., 2015, 33, 830–837 CrossRef CAS.
- H. Y. Tian, Z. P. Guo, J. Chen, L. Lin, J. L. Xia, X. Dong and X. S. Chen, Adv. Healthcare Mater., 2012, 1, 337–341 CrossRef CAS PubMed.
- J. Chen, H. Y. Tian, X. Dong, Z. P. Guo, Z. X. Jiao, F. F. Li, A. Kano, A. Maruyama and X. S. Chen, Macromol. Biosci., 2013, 13, 1438–1446 CrossRef CAS PubMed.
- M. J. Yang, S. H. Ku, D. Kim, W. J. Kim, H. Mok, S. H. Kim and I. C. Kwon, Macromol. Biosci., 2015, 15, 1755–1763 CrossRef CAS PubMed.
- U. Lungwitz, M. Breunig, T. Blunk and A. Gopferich, Eur. J. Pharm. Biopharm., 2005, 60, 247–266 CrossRef CAS PubMed.
- C. L. Fu, L. Lin, H. L. Shi, D. X. Zheng, W. Wang, S. Q. Gao, Y. F. Zhao, H. Y. Tian, X. J. Zhu and X. S. Chen, Biomaterials, 2012, 33, 4589–4596 CrossRef CAS PubMed.
- C. N. Xu, P. Wang, J. P. Zhang, H. Y. Tian, K. Park and X. S. Chen, Small, 2015, 11, 4321–4333 CrossRef CAS PubMed.
- H. Y. Tian, Z. H. Tang, X. L. Zhuang, X. S. Chen and X. B. Jing, Prog. Polym. Sci., 2012, 37, 237–280 CrossRef CAS.
- L. Zhao, J. X. Ding, C. S. Xiao, X. S. Chen, G. Q. Gai and L. Y. Wang, Acta Chim. Sin., 2015, 73, 60–65 CrossRef CAS.
- Y. Wu, W. Q. Chen, Y. Q. Zhao and H. R. Piao, Chin. Chem. Lett., 2015, 26, 334–338 CrossRef CAS.
Footnote |
† These authors contributed equally to this work. |
|
This journal is © the Partner Organisations 2017 |
Click here to see how this site uses Cookies. View our privacy policy here.