DOI:
10.1039/C7QM00302A
(Research Article)
Mater. Chem. Front., 2017,
1, 2283-2291
A family of ssa-type copper-based MOFs constructed from unsymmetrical diisophthalates: synthesis, characterization and selective gas adsorption†
Received
4th July 2017
, Accepted 10th August 2017
First published on 10th August 2017
Abstract
In this work, three porous MOFs (ZJNU-74, ZJNU-75 and ZJNU-76) were constructed via solvothermal reactions of unsymmetrical naphthalene-derived diisophthalate ligands and Cu2+ ions. As revealed by single-crystal X-ray diffraction analyses, they consist of dicopper [Cu2(COO)4] paddlewheels connected by the organic ligands to form three-dimensional networks with the same ssa topology, thus representing a MOF platform which allows understanding of the structure–property relationship. The gas adsorption properties of the three MOF compounds with respect to C2H2, CO2 and CH4 have been systematically investigated. They exhibit very high C2H2 uptakes varying from 179.2 to 188.6 cm3 (STP) g−1 and CO2 uptakes varying from 88.1 to 99.2 cm3 (STP) g−1 at 298 K and 1 atm. The predicted IAST selectivities range from 24.9 to 29.7 for an equimolar C2H2/CH4 gas mixture and from 4.8 to 5.4 for an equimolar CO2/CH4 gas mixture at 298 K and 1 atm. These results reveal that they possess very promising potential for C2H2/CH4 and CO2/CH4 separations. In particular, compared to the unmodified parent MOF ZJNU-74, the alkoxy group-functionalized MOFs ZJNU-75 and ZJNU-76 exhibit comparable and even higher C2H2 and CO2 uptake capacities as well as better C2H2/CH4 and CO2/CH4 adsorption selectivities under ambient conditions, which might be attributed to the optimized pore sizes as a result of ligand modification.
1. Introduction
Metal–organic frameworks (MOFs) formed by the assembly of inorganic metal nodes and organic ligands are emerging as compelling porous materials, which continue to attract enormous interest not only because of their intriguing architectures and topologies but also due to their potential applications in a wide range of fields including but not limited to gas adsorption/separation,1 luminescent sensors,2 heterogeneous catalysis,3 and drug delivery.4 Compared to traditional porous materials such as inorganic zeolites and activated carbons, MOFs can be rationally designed by selecting the diverse metal centres and the rich organic ligands, thus providing tailor-made pore structures and desired chemical behaviours. Also, MOFs tend to possess ordered crystal structures that can be determined by single-crystal/powder X-ray diffraction, which is conducive to their structure–property relationship elucidation and functional optimization. All of these attributes have created a scientific surge towards the discovery of new MOF materials for various applications.
In MOF synthesis, design and synthesis of organic ligands play a crucial role for targeting some new porous MOFs with desired structures and properties. Of various ligands, the aromatic multicarboxylate ligands are extensively studied because of the versatile coordination modes of the carboxylate groups and the rigidity of aromatic backbones capable of stabilizing the resulting frameworks. In particular, enormous efforts have been focused on the development of aromatic multicarboxylate building blocks incorporating isophthalate moieties for the construction of a range of MOFs because such an isophthalate moiety exhibits strong coordination ability and propensity to form polyhedral cages when reacting with metal ions under solvothermal conditions.5 For example, Schröder et al. reported a series of MOF materials derived from aromatic diisophthalate ligands for application in H2 storage.6 An rht-type MOF platform based on aromatic triisophthalate ligands was developed by different research groups,7 exhibiting impressive performance in the context of energy and environmental related gas storage of H2, CO2 and CH4. Inspired by these findings, we have been interested in the construction of porous MOFs based on the multicarboxylate ligands containing isophthalate moieties for various gas storage and separation applications. To date, we have reported a series of porous copper-based MOFs constructed from various linear/V-shaped diisophthalate linkers for C2H2 purification, CO2 capture, and methane storage.8 Furthermore, a literature survey showed that among the various multicarboxylates containing isophthalate moieties used for MOF fabrication, the symmetric and low-symmetric ones were frequently employed, while the unsymmetrical ones were less explored.9 This is presumably because the symmetric ligands are facilely synthesized and prone to crystallize in the self-assembly process. However, the use of unsymmetrical ligands in MOF construction has its own advantages of preventing the interpenetration of the resulting frameworks and engendering MOFs possessing novel topological structures.
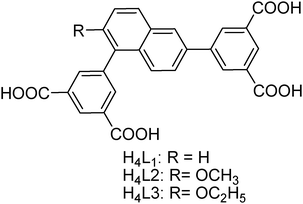 |
| Scheme 1 The chemical structures of the organic ligands used to construct ZJNU-74, ZJNU-75 and ZJNU-76. | |
Acetylene plays a very important role in daily life and the modern chemical industry. For its widespread use, two issues need to be addressed. One issue we have to face is concerning acetylene storage which is very challenging because it cannot be stored in a steel cylinder under high pressure like other fuel gases such as H2 and CH4 due to its highly explosive nature. Another issue is regarding its production where the separation of acetylene from other gases which include CO2 and CH4 is needed. In addition, the separation of CO2 from natural gas is also very important from an environmental and energy perspective because CO2 as an undesirable impurity in natural gas not only reduces the energy content of the natural gas but also causes pipeline corrosion in the presence of water. Among the various techniques proposed to address the above-mentioned issues, the adsorption method is considered as one of the most promising and applicable approaches owning to its simple operation, effectiveness and low cost. The key lies in finding efficient porous materials. In this regard, MOFs have been considered as good candidates due to their prominent characteristics of modular synthetic routes, high crystalline nature, and tuneable structures and properties as well as their easily functionalized interior pores.
On the basis of the above considerations and as a continuation of our investigation, we designed and synthesized three unsymmetrical diisophthalate ligands consisting of two terminal isophthalate moieties connected to the 1,6 position of a central naphthyl core (Scheme 1). As demonstrated in the literature,5c,10 an alkoxy group as a common polar group can improve gas adsorption properties via electric and/or steric effects. Therefore, the central naphthyl core is functionalized with different alkoxy groups including methoxy and ethoxy groups to tune the gas adsorption behaviours. With these ligands in hand, we successfully constructed three copper-based MOFs under solvothermal conditions. Furthermore, their gas adsorption properties with respect to C2H2, CO2 and CH4 were systematically investigated, revealing that they possess promising potential for C2H2 storage and purification as well as natural gas purification. In this work, we wish to report the solvothermal syntheses, crystal structures and selective gas adsorption properties of the three resulting MOF compounds.
2. Experimental
2.1 Materials and measurements
1,6-Dibromonaphthalene,11 1,6-dibromo-2-methoxy naphthalene,12 1,6-dibromo-2-ethoxynaphthalene,12 and dimethyl 5-(pinacolboryl)isophthalate8i were synthesized according to the published procedures. All other chemical reagents were obtained from commercial sources and used as received without further purification. The solution 1H and 13C NMR (Nuclear Magnetic Resonance) spectra were recorded on Bruker AV400 or AV600 instruments. The chemical shifts (δ values) were recorded in ppm (parts per million) related to the residual solvent peaks and the coupling constants (J values) were reported in Hz. The FTIR (Fourier Transform Infrared) spectra were recorded in transmission mode in the range of 4000–400 cm−1 using a Nicolet 5DX FTIR spectrometer with KBr pellets. Elemental analyses (C, H, and N) were acquired on a Perkin-Elmer 240 CHN elemental analyser. Powder X-ray diffraction (PXRD) data were collected at room temperature with a Philips PW3040/60 automated diffractometer using monochromatic Cu-Kα radiation (λ = 1.5418 Å). The simulated powder patterns were calculated using Mercury 1.4.1 based on X-ray crystal structures of the corresponding MOFs. Thermogravimetric analysis (TGA) was carried out on a Netzsch STA 449C thermal analyser in flowing nitrogen with a heating rate of 5 K min−1. Gas adsorption isotherms were measured using a Micromeritics ASAP 2020HD88 surface-area-and-porosity analyser. The temperature of 77 K was maintained using liquid nitrogen, and other temperatures were controlled by means of a circulating bath (Julabo, F12ED). Highly pure gases (C2H2, 99.9%; CH4, 99.99%; CO2, 99.999%) were used for the measurements.
2.2 Single-crystal data collections
Single-crystal X-ray diffraction data were collected on a Bruker SMART APEX II CCD diffractometer equipped with a graphite-monochromated Mo-Kα radiation (λ = 0.71073 Å) and Cu-Kα radiation source (λ = 1.5418 Å). The structures were solved by direct methods, and the refinements have been made using full-matrix least squares methods based on F2 with the SHELXTL package. All non-hydrogen atoms were found from the Fourier difference maps and refined anisotropically. The hydrogen atom positions were fixed geometrically at calculated distances and allowed to ride on the parent atoms. During the refinement, diffused electron densities resulting from disordered solvent molecules were removed from the dataset using the SQUEEZE routine of PLATON.13 The resulting new sets of hkl reflections were used for further structure determination and refinement. Crystallographic data and structure refinement parameters are listed in Table S4 in the ESI.† CCDC 1558048, 1558537 and 1543094 contain the supplementary crystallographic data for ZJNU-74, ZJNU-75 and ZJNU-76.†
2.3 Synthesis and characterization of the organic ligands
5,5′-(2-Methoxy-naphthyl-1,6-diyl)diisophthalate (H4L2).
1,6-Dibromo-2-methoxynaphthalene (1.00 g, 3.16 mmol), methyl 5-(pinacolboryl)isophthalate (2.23 g, 6.96 mmol), Cs2CO3 (3.09 g, 9.49 mmol) and tetrakis(triphenylphosphine)palladium (0.18 g, 0.16 mmol) were added to a 250 mL single-necked round-bottom flask equipped with a magnetic stirrer and a condenser. The flask was evacuated and back-filled with nitrogen. After the process was repeated three times, dry dioxane (80 mL) was added to the flask via a syringe. The resulting mixture was stirred under a nitrogen atmosphere, and heated under reflux for 72 h. After the mixture was allowed to cool to room temperature, the solvent was removed under reduced pressure. The residue was extracted with dichloromethane (80 mL × 3). The combined extracts were washed with brine (80 mL) and dried over anhydrous magnesium sulphate, and the solvent was removed under vacuum. Recrystallization with toluene followed by purification by silica gel column chromatography eluting with petroleum ether/dichloromethane/ethyl acetate (10/10/1, v/v/v) gave the tetramethyl ester intermediate as an off-white solid (0.81 g, 1.49 mmol, 47% yield). 1H NMR (CDCl3, 400.1 MHz) δ (ppm): 8.805 (t, J = 1.6 Hz, 1H), 8.689 (t, J = 1.6 Hz, 1H), 8.589 (d, J = 1.6 Hz, 2H), 8.304 (d, J = 1.6 Hz, 2H), 8.163 (d, J = 1.6 Hz, 1H), 8.051 (d, J = 9.2 Hz, 1H), 7.695 (dd, J = 9.2 Hz, 2.0 Hz, 1H), 7.497 (d, J = 9.2 Hz, 1H), 7.463 (d, J = 9.2 Hz, 1H), 4.017 (s, 6H), 3.987 (s, 6H), 3.895 (s, 3H).
To a suspension of the tetramethyl ester (0.81 g, 1.49 mmol) in THF (30 mL) and methanol (30 mL) was added 6 M NaOH (30 mL, 180 mmol). The resulting mixture was stirred under reflux for 12 h. After it was cooled to room temperature, the solvent was removed under vacuum. The residue was dissolved in water and acidified using concentrated HCl under ice-water bath. The solid was collected by filtration, washed with water, and dried under vacuum at 343 K to give the target compound as an off-white solid in 98% yield (0.71 g, 1.46 mmol). The H4L2 ligand was obtained in an overall yield of 46% based on the starting materials. 1H NMR (DMSO-d6, 400.1 MHz) δ (ppm): 8.569 (t, J = 1.6 Hz, 1H), 8.512 (d, J = 1.6 Hz, 2H), 8.478 (t, J = 1.6 Hz, 1H), 8.419 (d, J = 1.6 Hz, 1H), 8.261 (d, J = 9.2 Hz, 1H), 8.079 (d, J = 1.6 Hz, 2H), 7.821 (dd, J = 1.6 Hz, 9.2 Hz, 1H), 7.664 (d, J = 9.2 Hz, 1H), 7.416 (d, J = 9.2 Hz, 1H), 3.861 (s, 3H); 13C NMR (DMSO-d6, 150.9 MHz) δ (ppm): 167.140, 154.625, 141.071, 137.093, 135.985, 133.818, 132.924, 132.518, 132.316, 131.591, 131.304, 129.407, 129.217, 126.764, 126.330, 125.361, 122.279, 114.935, 56.887; selected FTIR (KBr, cm−1): 1701, 1599, 1489, 1450, 1255, 1068, 758, 669.
5,5′-(2-Ethoxy-naphthyl-1,6-diyl)diisophthalate (H4L3).
The synthetic procedure of H4L3 was similar to that of H4L2 except that 1,6-dibromo-2-ethoxynaphthalene was used instead of 1,6-dibromo-2-methoxynaphthalene. The overall yield is about 52%. 1H NMR (DMSO-d6, 600.1 MHz) δ (ppm): 8.547 (t, J = 1.2 Hz, 1H), 8.468 (t, J = 1.2 Hz, 1H), 8.449 (d, J = 1.2 Hz, 2H), 8.354 (d, J = 1.2 Hz, 1H), 8.196 (d, J = 9.0 Hz, 1H), 8.047 (d, J = 1.2 Hz, 2H), 7.788 (dd, J = 9.0 Hz, 1.2 Hz, 1H), 7.610 (d, J = 9.0 Hz, 1H), 7.453 (d, J = 9.0 Hz, 1H), 4.174 (q, J = 7.2 Hz, 2H), 1.175 (t, J = 7.2 Hz, 3H); 13C NMR (DMSO-d6, 150.19 MHz) δ (ppm): 166.453, 153.945, 139.314, 138.661, 133.824, 133.717, 132.461, 132.069, 131.303, 129.931, 129.349, 129.228, 128.938, 126.828, 126.295, 125.391, 122.869, 116.420, 65.128, 15.149; selected FTIR (KBr, cm−1): 1701, 1597, 1448, 1248, 1063, 756, 669.
5,5′-(Naphthyl-1,6-diyl)diisophthalate (H4L1).
The synthetic procedure of H4L1 is analogous to that of H4L2, except that 1,6-dibromonaphthalene was used instead of 1,6-dibromo-2-methoxynaphthalene. The overall yield is about 49%. 1H NMR (DMSO-d6, 600.1 MHz) δ (ppm): 8.599 (t, J = 1.8 Hz, 1H), 8.564 (d, J = 1.2 Hz, 2H), 8.517 (t, J = 1.8 Hz, 1H), 8.505 (d, J = 1.8 Hz, 1H), 8.252 (d, J = 1.8 Hz, 2H), 8.216 (d, J = 8.4 Hz, 1H), 7.953 (dd, J = 9.0 Hz, 1.8 Hz, 1H), 7.873 (d, J = 9.0 Hz, 1H), 7.693 (t, J = 8.4 Hz, 1H), 7.582 (dd, J = 7.2 Hz, 1.2 Hz, 1H); 13C NMR (DMSO-d6, 150.9 MHz) δ (ppm): 167.088, 167.036, 140.936, 137.931, 136.365, 134.658, 134.278, 132.948, 132.515, 131.896, 130.540, 129.606, 129.567, 129.527, 128.307, 127.069, 126.807, 126.299, 126.209; selected FTIR (KBr, cm−1): 1707, 1601, 1406, 1265, 1232, 1144, 1068, 762, 669.
2.4 Synthesis and characterization of the MOF compounds
ZJNU-74: a mixture of H4L1 (5.0 mg, 11.0 μmol) and Cu(NO3)2·3H2O (15.0 mg, 62.1 μmol) was dissolved in N,N-dimethylformamide (DMF, 1.5 mL) in a 20 mL glass vial. After 0.3 mL of HBF4 (48%) was added, the vial was capped and placed in an oven preheated to 353 K. After 48 h, the blue hexagon-shaped crystals that formed were separated while the mother liquor was still warm and washed with fresh DMF. The yield is ca. 46% based on the organic ligand. Based on the single-crystal X-ray diffraction determination, TGA (Fig. S2a, ESI†) and microanalyses, ZJNU-74 can be best formulated as [Cu2(L1)(H2O)2]·4DMF·2H2O. Elemental analysis for C38H48N4O16Cu2, calcd: C, 48.35%, H, 5.13%, N, 5.94%; found: C, 48.39%, H, 5.19%, N, 5.82%. Selected FTIR (KBr, cm−1): 1655, 1578, 1437, 1371, 1304, 1254, 1101, 777, 729.
ZJNU-75: a mixture of Cu(NO3)2·3H2O (15.0 mg, 62.1 μmol) and the organic ligand H4L2 (5.0 mg, 10.3 μmol) was dissolved in DMF (1.5 mL) in a 20 mL glass vial. After the addition of HBF4 (48%, 0.45 mL), the vial was capped tightly and placed in an oven which has been preheated at 363 K. After 24 h, the blue hexagonal-shaped crystals produced were collected by filtration when the mother liquor was still warm. The yield is about 34% based on the organic ligands. Based on the single-crystal X-ray diffraction structural determination, TGA (Fig. S2b, ESI†) and microanalyses, ZJNU-75 can be best formulated to be [Cu2(L2)(H2O)2]·5DMF·H2O. Elemental analysis for C42H55N5O17Cu2, calcd: C, 49.02%, H, 5.39%, N, 6.81%; found: C, 49.11%, H, 5.28%, N, 6.84%; selected FTIR (KBr, cm−1): 1655, 1578, 1437, 1371, 1257, 1101, 1065, 771, 729, 488.
ZJNU-76: 1.5 mL of DMF was added to a 20 mL glass vial containing the organic ligand H4L3 (5.0 mg, 10.0 μmol) and Cu(NO3)2·3H2O (5.0 mg, 20.7 μmol). After the addition of 0.3 mL of HBF4 (48%), the vial was capped tightly and placed in an oven preheated to 333 K. After the reaction time of 36 h, the blue hexagonal-shaped crystals were isolated by filtration when the mother liquor was still warm. The yield is about 43% based on the organic ligand. Based on the single-crystal X-ray diffraction structural determination, TGA (Fig. S2c, ESI†) and microanalyses, ZJNU-76 can be best formulated to be [Cu2(L3)(H2O)2]·5DMF·H2O. Elemental analysis for C43H57N5O17Cu2, calcd: C, 49.52%, H, 5.51%, N, 6.71%; found: C, 49.46%, H, 5.42%, N, 6.74%; selected FTIR (KBr, cm−1): 1655, 1585, 1437, 1371, 1254, 1101, 1059, 773, 729, 488.
3. Results and discussion
3.1 Synthesis and characterization
The organic ligands were readily synthesized through hydrolysis of the corresponding tetramethyl esters which were prepared by Suzuki–Miyaura cross-coupling reactions between dimethyl 5-(pinacolboryl)isophthalate and the corresponding dibromo compounds using Pd(PPh3)4 as a catalyst and Cs2CO3 as a base in dioxane. The chemical structures of the organic ligands have been confirmed by means of 1H NMR, 13C NMR and FTIR spectra. The combination of the organic ligands and Cu(NO3)2·3H2O under solvothermal reaction conditions afforded blue hexagon-shaped crystals of ZJNU-74 (based on H4L1), ZJNU-75 (based on H4L2) and ZJNU-76 (based on H4L3). Their structures were characterized by single-crystal X-ray diffraction and the phase purity of the bulk materials was evidenced by a good agreement between their experimental and simulated PXRD patterns (Fig. S1, ESI†).
3.2 Structure descriptions
Single-crystal X-ray diffraction studies indicate that the three MOF compounds are isostructural. Therefore, only the crystal structure of compound ZJNU-74 as a representative is described in detail. The MOF compound ZJNU-74 crystallizes in the hexagonal space group of P63/mmc with unit cell parameters of a = b = 18.3150(7) Å, c = 26.7665(14) Å, α = β = 90°, and γ = 120°. Apart from the guest molecules, the asymmetric unit consists of half of a crystallographically independent Cu2+ ion, one quarter of an independent deprotonated ligand and half of a coordinated water molecule. As for metal ions, each copper ion displays a pentacoordinated [CuO5] square-pyramidal coordination geometry which is surrounded by four carboxylate oxygen atoms from four different organic linker anions in an equatorial plane and one oxygen atom from a terminal water molecule in an apical position. The copper–Ocarboxylate bond distance varies from 1.932 to 1.938 Å, which is well comparable to those commonly reported in the literature for copper–carboxylate complexes.14 Two adjacent copper ions are bridged through four carboxylate groups to form a typical dicopper paddlewheel unit with a Cu–Cu distance of 2.676 Å. As for the organic ligands, four carboxylate groups are fully deprotonated and involved in coordination with copper ions in a bidentate μ2:η1η1 coordination fashion. It is noteworthy to point out that the organic ligand is incorporated into the framework only in one conformation, which is different from the cases occurring in most of the other copper-based MOFs constructed from bent diisophthalate ligands where the organic ligands usually adopt two different conformations in the network structures.5i,15 Moreover, the dicopper paddlewheel units are further connected to each other by the organic linkers, thereby producing a three-dimensional (3D) network. The most striking structure feature is that three different types of metal–organic cages as well as two types of one-dimensional channels with different sizes coexist in the network. As shown in Fig. 1, cage A is composed of six dicopper paddlewheels and three organic ligands (Fig. 1b) with a dimension of about 4 × 9 Å, taking into account the van der Waals radii (the same as below). Cage B with a diameter of around 11 Å is constructed by six dicopper paddlewheels, six isophthalate moieties and six organic ligands (Fig. 1c). Cage C with dimensions of 6 × 9 Å is defined by six dicopper paddlewheels and six half organic ligands (Fig. 1d). In addition to hierarchical cages, two different types of channels can be also observed viewing along the crystallographic c axis. One channel is formed by alternatively packing cage A and cage B via sharing triangular windows built of three dicopper paddlewheels, while another channel is generated by stacking cage C via sharing the central naphthyl moieties of the organic linkers. In order to simplify the 3D framework structure, the topological analysis approach was performed. The dicopper paddlewheel units are connected to four organic ligands and therefore can be regarded as four-connected nodes. Also, the organic ligands are connected to four dicopper paddlewheel units and therefore can be considered as 4-connected nodes. On the basis of this simplification, the structure of ZJNU-74 can be assigned to be a binodal 4-connected 3D framework with ssa topology (Schläfli symbol: {42·64}{42·84}), as analysed by the Topos 4.0 program.
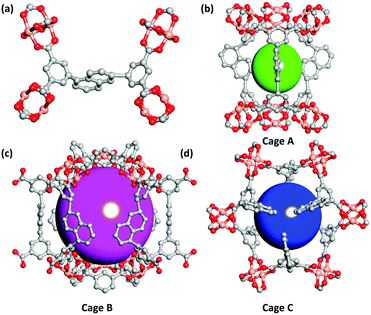 |
| Fig. 1 (a) The coordination environment of the organic linker and (b–d) three types of polyhedral cages with different shapes and sizes in ZJNU-74. Hydrogen atoms and coordinated water molecules are omitted for clarity. | |
3.3 Permanent porosity
The porous properties of three MOF compounds were investigated by N2 adsorption–desorption experiments performed at 77 K. Prior to gas adsorption measurements, the as-synthesized samples were solvent-exchanged with dry acetone multiple times followed by evacuation under dynamic vacuum at 373 K until the degassed rate reached 2 μmHg per min. As shown in Fig. 2a, the three MOF compounds exhibited typical Type-I adsorption isotherms with a steep increase of N2 uptake in the low-pressure region followed by a plateau, which is a characteristic of a microporous structure. Based on the N2 isotherms, the Brunauer–Emmett–Teller (BET) and Langmuir surface areas are evaluated to be 2243 and 2488 m2 g−1 for ZJNU-74, 2063 and 2279 m2 g−1 for ZJNU-75, and 1996 and 2256 m2 g−1 for ZJNU-76 (Fig. S4–S6, ESI†). These values are among the highest reported for porous copper-based MOFs based on V-shaped diisophthalate ligands.10c,15,16 The smaller surface areas of MOFs ZJNU-75 and ZJNU-76 compared to their parent MOF ZJNU-74 are due to the alkoxy groups attached to the organic ligands occupying the pore space. The total pore volumes calculated from the maximum amounts of N2 adsorption are 0.8878 cm3 g−1 for ZJNU-74, 0.8147 cm3 g−1 for ZJNU-75 and 0.8048 cm3 g−1 for ZJNU-76. Also, the pore size distributions were calculated using nonlocal density functional theory (NLDFT). They all present two distinct pore sizes centred at 5.90 and 8.58 Å for ZJNU-74, 5.90 and 8.04 Å for ZJNU-75, and 5.36 and 8.04 Å for ZJNU-76, which are consistent with the single-crystal X-ray structure analyses.
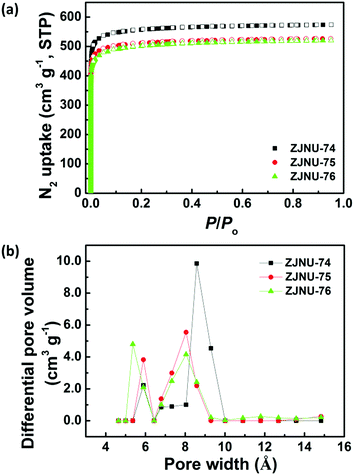 |
| Fig. 2 (a) N2 adsorption–desorption isotherms at 77 K and (b) pore size distributions (determined by the NLDFT method) of three MOF compounds investigated. The solid and open symbols represent adsorption and desorption, respectively. STP = standard temperature and pressure. | |
3.4 Selective C2H2/CH4 and CO2/CH4 separations
Establishment of permanent porosity encourages us to explore their potential in C2H2/CH4 and CO2/CH4 gas separations, which are relevant to C2H2 storage and purification as well as natural gas purification. For fulfilling this purpose, C2H2, CO2, and CH4 isotherms were measured at three different temperatures of 278 K, 288 K and 298 K and pressures up to 1 atm (Fig. 3). The three MOF compounds exhibit high C2H2 adsorption capacities. At 298 K and 1 atm, the C2H2 uptakes reach 180.0, 188.6 and 179.2 cm3 (STP) g−1 for ZJNU-74, ZJNU-75 and ZJNU-76, respectively, which increase to 242.3, 240.4 and 227.2 cm3 (STP) g−1, respectively when the temperature is lowered to 278 K. The C2H2 uptakes of the three MOF materials are higher than those of most reported MOFs such as MOF-5 (26 cm3 (STP) g−1),17 UTSA-33 (83.6 cm3 (STP) g−1),18 BUT-70B (87.1 cm3 (STP) g−1),19 [Zn2(NDC)2(dabco)] (106 cm3 (STP) g−1),20 MIL-100(Fe) (119 cm3 (STP) g−1),21 UTSA-34b (120.8 cm3 (STP) g−1),14b NOTT-102 (146.3 cm3 (STP) g−1),22 MOF-505 (148 cm3 (STP) g−1),17 Cu-TDPAH (155.7 cm3 (STP) g−1),23 Cu-EBTC (160 cm3 (STP) g−1),24 {[Co6(OH)2(INA)6(CPT)3](NO3)} (163 cm3 (STP) g−1),25 Cu-DDC (164 cm3 (STP) g−1)26 and CPM-231 (177.6 cm3 (STP) g−1),27 and only lower than those of several top-performing MOF materials such as ZJNU-47 (213 cm3 (STP) g−1),8e NJU-Bai17 (222.4 cm3 (STP) g−1),28 FJI-H8 (224 cm3 (STP) g−1),5c MFM-188 (232 cm3 (STP) g−1)29 and ZJU-12 (244 cm3 (STP) g−1).10a Notably, the alkoxy group-functionalized MOFs ZJNU-75 and ZJNU-76 exhibited comparable and even higher C2H2 uptakes under ambient conditions compared to their unmodified parent MOF ZJNU-74, despite their lower surface areas and pore volumes. This might be attributed to the effect of pore size playing a more important role than the effect of surface area and pore volume. The narrow pore sizes in the alkoxy-functionalized frameworks enhance the affinity toward C2H2 molecules due to the overlapping attractive potential from the pore walls. The C2H2 storage density in the bulk material of ZJNU-75 at 298 K and 1 atm reached 0.171 g cm−3, which is equivalent to the density of acetylene at 16.2 MPa at 298 K, and is 81.2 times larger than the value of the compression limit (0.2 MPa) for the safe storage of acetylene at room temperature. Besides, the three MOF compounds also display high CO2 adsorption capacities. At 298 K and 1 atm, the CO2 uptakes are 88.1, 99.2 and 95.8 cm3 (STP) g−1 for ZJNU-74, ZJNU-75 and ZJNU-76, respectively, which increase to 148.1, 164.2, and 158.9 cm3 (STP) g−1, respectively when the temperature is lowered to 278 K. These values are also among the highest reported for porous copper-based MOFs constructed from V-shaped diisophthalate ligands.15a,16b,c
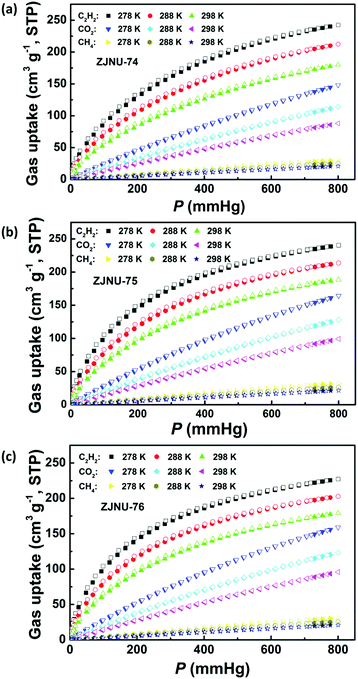 |
| Fig. 3 C2H2, CO2 and CH4 isotherms of (a) ZJNU-74, (b) ZJNU-75, and (c) ZJNU-76 at three different temperatures of 278 K, 288 K and 298 K. The solid and open symbols represent adsorption and desorption, respectively. | |
In sharp contrast, the three MOF compounds take up a very limited amount of CH4. At 298 K and 1 atm, the CH4 uptakes are 20.8, 21.6 and 21.0 cm3 (STP) g−1 for ZJNU-74, ZJNU-75 and ZJNU-76, respectively, which are systematically lower than the corresponding C2H2 and CO2 uptakes, indicating their promising potential for C2H2/CH4 and CO2/CH4 separations. To evaluate their separation performance, we calculated the adsorption selectivity based on the pure-component isotherms according to ideal adsorption solution theory (IAST). The IAST calculation results for the equimolar C2H2–CH4 and CO2–CH4 gas mixtures are presented in Fig. 4a and b. For all three MOF compounds, the IAST C2H2/CH4 selectivities decrease with the increase of pressure, while the IAST CO2/CH4 selectivities slightly increase as the pressure increases. At 298 K and 1 atm, the C2H2/CH4 IAST selectivities are 24.9, 28.2 and 29.7 for ZJNU-74, ZJNU-75 and ZJNU-76, respectively, which come up to 31.6, 36.1 and 38.2, respectively when the temperature decreases to 278 K (Fig. S10–S12, ESI†). The C2H2/CH4 adsorption selectivity of ZJNU-76 at 298 K and 1 atm exceeds those of some reported MOF materials such as ZJU-11 (19),30 Zn5(BTA)6(TDA)2 (15.5),31 Ni-TATB (14.1),32 Zn2(NH2-BTB)(2-nim) (8),33 and UTSA-10 (4.3).34 For the equimolar CO2/CH4 gas mixture, the IAST selectivities are 4.80, 5.44 and 5.43 for ZJNU-74, ZJNU-75 and ZJNU-76, respectively at 298 K and 1 atm, which come up to 6.09, 6.98 and 7.14 at 278 K (Fig. S10–S12, ESI†). The CO2/CH4 adsorption selectivity of ZJNU-76 at 298 K and 1 atm is comparable to and even higher than those of some reported copper-based MOF compounds based on V-shaped diisophthalate linkers such as Cu2L (5.4, L = 5,5′-carbonyldiisophthalic acid)16a and PCN-88 (3.8).5g The preferable adsorption of C2H2 and CO2 over CH4 may be attributed to the smaller kinetic diameters of CO2 (3.3 Å) and C2H2 (3.3 Å) compared to CH4 (3.8 Å), as well as much stronger interactions between C2H2/CO2 and the frameworks as indicated by the data of isosteric heat of adsorption calculated using the Clausius–Claperyron equation. As shown in Fig. 4d, the isosteric heats of C2H2 and CO2 adsorption are significantly higher than that of CH4 adsorption from low to high coverages. In particular, by comparison, the alkoxy group-functionalized MOFs ZJNU-75 and ZJNU-76 exhibited higher C2H2/CH4 and CO2/CH4 adsorption selectivities than their parent MOF compound ZJNU-74, which might be attributed to the optimized pore sizes as a result of ligand modification. Besides, the selective C2H2/CO2 adsorption properties of the three MOFs investigated were also evaluated, revealing that they exhibit a comparable C2H2/CO2 adsorption selectivity (Fig. 4c and Fig. S10–S12, ESI†). The predicted C2H2/CO2 IAST adsorption selectivity for an equimolar C2H2–CO2 mixture at 298 K and 1 atm is 4.4, 4.4 and 4.7 for ZJNU-74, ZJNU-75 and ZJNU-76, respectively. When the temperature is lowered to 278 K, the corresponding values turn out to be 4.1, 4.0 and 4.2. Overall, the above results indicate that the three MOF compounds serve as an excellent platform having prospective applications in the separation and purification of C2H2 and natural gas, and their gas separation properties can be modulated/improved by ligand functionalization.
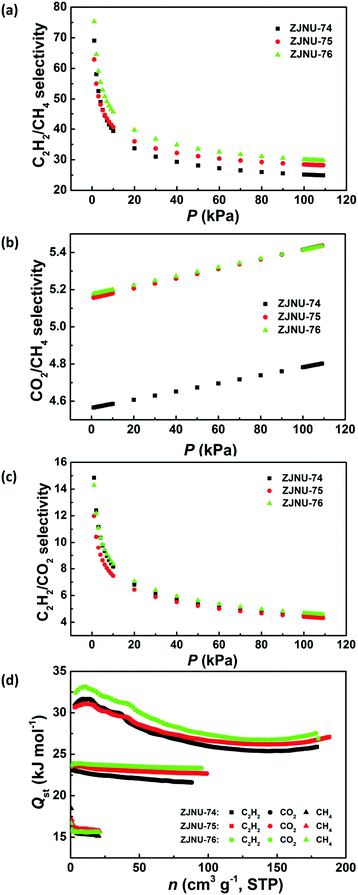 |
| Fig. 4 The IAST selectivity for the equimolar (a) C2H2/CH4, (b) CO2/CH4 and (c) C2H2/CO2 gas mixtures at 298 K in three MOF compounds investigated. (d) The isosteric heat of C2H2, CO2 and CH4 adsorption in three MOF compounds investigated as a function of gas loading. | |
4. Conclusions
In summary, three unsymmetrical naphthalene-derived diisophthalate ligands were designed and synthesized for the first time to construct porous copper-based MOFs. Irrespective of their unsymmetric structures, the resulting three MOF compounds display the same ssa topological structure as revealed by single-crystal X-ray diffraction studies. With moderate surface area and suitable pore space, they exhibited promising potential for C2H2/CH4 and CO2/CH4 separations. Furthermore, by ligand modification with different alkoxy groups, their pore sizes and physicochemical properties and thus gas adsorption properties can be modulated. In particular, compared to the parent compound, the alkoxy group-modified MOF compounds exhibit comparable and even higher C2H2 and CO2 uptake capacities as well as higher C2H2/CH4 and CO2/CH4 adsorption selectivities under ambient conditions. These findings open up avenues for the design and synthesis of porous MOFs from unsymmetrical multiisophthalate ligands for various applications.
Conflicts of interest
There are no conflicts to declare.
Acknowledgements
We gratefully acknowledge the Natural Science Foundation of Zhejiang province, China (LR16B010001), the National Natural Science Foundation of China (No. 21301156), and the Qianjiang Talents Project in Zhejiang province (ZC304015017) for generous financial support.
References
-
(a) M. Gehre, Z. Guo, G. Rothenberg and S. Tanase, ChemSusChem, 2017 DOI:10.1002/cssc.201700657;
(b) K. Adil, Y. Belmabkhout, R. S. Pillai, A. Cadiau, P. M. Bhatt, A. H. Assen, G. Maurin and M. Eddaoudi, Chem. Soc. Rev., 2017, 46, 3402–3430 RSC;
(c) A. Schoedel, Z. Ji and O. M. Yaghi, Nat. Energy, 2016, 1, 16034 CrossRef CAS;
(d) Y. He, W. Zhou, G. Qian and B. Chen, Chem. Soc. Rev., 2014, 43, 5657–5678 RSC;
(e) Z. Zhang, Z.-Z. Yao, S. Xiang and B. Chen, Energy Environ. Sci., 2014, 7, 2868–2899 RSC;
(f) T. A. Makal, J.-R. Li, W. Lu and H.-C. Zhou, Chem. Soc. Rev., 2012, 41, 7761–7779 RSC;
(g) Y. He, W. Zhou, R. Krishna and B. Chen, Chem. Commun., 2012, 48, 11813–11831 RSC;
(h) J.-P. Zhang, Y.-B. Zhang, J.-B. Lin and X.-M. Chen, Chem. Rev., 2012, 112, 1001–1033 CrossRef CAS PubMed;
(i) H. Wu, Q. Gong, D. H. Olson and J. Li, Chem. Rev., 2012, 112, 836–868 CrossRef CAS PubMed;
(j) M. P. Suh, H. J. Park, T. K. Prasad and D.-W. Lim, Chem. Rev., 2012, 112, 782–835 CrossRef CAS PubMed;
(k) J.-R. Li, J. Sculley and H.-C. Zhou, Chem. Rev., 2012, 112, 869–932 CrossRef CAS PubMed;
(l) K. Sumida, D. L. Rogow, J. A. Mason, T. M. McDonald, E. D. Bloch, Z. R. Herm, T.-H. Bae and J. R. Long, Chem. Rev., 2012, 112, 724–781 CrossRef CAS PubMed;
(m) R. B. Getman, Y.-S. Bae, C. E. Wilmer and R. Q. Snurr, Chem. Rev., 2012, 112, 703–723 CrossRef CAS PubMed;
(n) S. Ma and H.-C. Zhou, Chem. Commun., 2010, 46, 44–53 RSC.
-
(a) W. P. Lustig, S. Mukherjee, N. D. Rudd, A. V. Desai and J. L. S. K. Ghosh, Chem. Soc. Rev., 2017, 46, 3242–3285 RSC;
(b) Z. Hu, B. J. Deibert and J. Li, Chem. Soc. Rev., 2014, 43, 5815–5840 RSC;
(c) Y. Cui, Y. Yue, G. Qian and B. Chen, Chem. Rev., 2012, 112, 1126–1162 CrossRef CAS PubMed;
(d) L. E. Kreno, K. Leong, O. K. Farha, M. Allendorf, R. P. V. Duyne and J. T. Hupp, Chem. Rev., 2012, 112, 1105–1125 CrossRef CAS PubMed;
(e) M. D. Allendorf, C. A. Bauer, R. K. Bhakta and R. J. T. Houk, Chem. Soc. Rev., 2009, 38, 1330–1352 RSC.
-
(a) M. Zhao, S. Ou and C.-D. Wu, Acc. Chem. Res., 2014, 47, 1199–1207 CrossRef CAS PubMed;
(b) M. Yoon, R. Srirambalaji and K. Kim, Chem. Rev., 2012, 112, 1196–1231 CrossRef CAS PubMed;
(c) G. Nickerl, A. Henschel, R. Grünker, K. Gedrich and S. Kaskel, Chem. Ing. Tech., 2011, 83, 90–103 CrossRef CAS;
(d) A. Corma, H. García and F. X. L. i. Xamena, Chem. Rev., 2010, 110, 4606–4655 CrossRef CAS PubMed;
(e) J. Lee, O. K. Farha, J. Roberts, K. A. Scheidt, S. T. Nguyen and J. T. Hupp, Chem. Soc. Rev., 2009, 38, 1450–1459 RSC.
-
(a) P. Horcajada, R. Gref, T. Baati, P. K. Allan, G. Maurin, P. Couvreur, G. Férey, R. E. Morris and C. Serre, Chem. Rev., 2012, 112, 1232–1268 CrossRef CAS PubMed;
(b) P. Horcajada, T. Chalati, C. Serre, B. Gillet, C. Sebrie, T. Baati, J. F. Eubank, D. Heurtaux, P. Clayette, C. Kreuz, J.-S. Chang, Y. K. Hwang, V. Marsaud, P.-N. Bories, L. Cynober, S. Gil, G. Férey, P. Couvreur and R. Gref, Nat. Mater., 2010, 9, 172–178 CrossRef CAS PubMed.
-
(a) M. Zhang, X. Xin, Z. Xiao, R. Wang, L. Zhang and D. Sun, J. Mater. Chem. A, 2017, 5, 1168–1175 RSC;
(b) I. Spanopoulos, C. Tsangarakis, E. Klontzas, E. Tylianakis, G. Froudakis, K. Adil, Y. Belmabkhout, M. Eddaoudi and P. N. Trikalitis, J. Am. Chem. Soc., 2016, 138, 1568–1574 CrossRef CAS PubMed;
(c) J. Pang, F. Jiang, M. Wu, C. Liu, K. Su, W. Lu, D. Yuan and M. Hong, Nat. Commun., 2015, 6, 7575 CrossRef PubMed;
(d) T. K. Pal, D. De, S. Neogi, P. Pachfule, S. Senthilkumar, Q. Xu and P. K. Bharadwaj, Chem. – Eur. J., 2015, 21, 19064–19070 CrossRef CAS PubMed;
(e) Y. He, B. Li, M. O'Keeffe and B. Chen, Chem. Soc. Rev., 2014, 43, 5618–5656 RSC;
(f) J. Pang, F. Jiang, M. Wu, D. Yuan, K. Zhou, J. Qian, K. Su and M. Hong, Chem. Commun., 2014, 50, 2834–2836 RSC;
(g) J.-R. Li, J. Yu, W. Lu, L.-B. Sun, J. Sculley, P. B. Balbuena and H.-C. Zhou, Nat. Commun., 2013, 4, 1538 CrossRef PubMed;
(h) Y.-S. Xue, Y. He, S.-B. Ren, Y. Yue, L. Zhou, Y.-Z. Li, H.-B. Du, X.-Z. You and B. Chen, J. Mater. Chem., 2012, 22, 10195–10199 RSC;
(i) D. Frahm, M. Fischer, F. Hoffmann and M. Fröba, Inorg. Chem., 2011, 50, 11055–11063 CrossRef CAS PubMed;
(j) P. Zhang, B. Li, Y. Zhao, X. Meng and T. Zhang, Chem. Commun., 2011, 47, 7722–7724 RSC;
(k) X. Lin, J. Jia, X. Zhao, K. M. Thomas, A. J. Blake, G. S. Walker, N. R. Champness, P. Hubberstey and M. Schröder, Angew. Chem., Int. Ed., 2006, 45, 7358–7364 CrossRef CAS PubMed;
(l) B. Chen, N. W. Ockwig, A. R. Millward, D. S. Contreras and O. M. Yaghi, Angew. Chem., Int. Ed., 2005, 44, 4745–4749 CrossRef CAS PubMed.
- Y. Yan, S. Yang, A. J. Blake and M. Schröder, Acc. Chem. Res., 2014, 47, 296–307 CrossRef CAS PubMed.
-
(a) R. Luebke, Ł. J. Weseliński, Y. Belmabkhout, Z. Chen, Ł. Wojtas and M. Eddaoudi, Cryst. Growth Des., 2014, 14, 414–418 CrossRef CAS;
(b) C. E. Wilmer, O. K. Farha, T. Yildirim, I. Eryazici, V. Krungleviciute, A. A. Sarjeant, R. Q. Snurr and J. T. Hupp, Energy Environ. Sci., 2013, 6, 1158–1163 RSC;
(c) X.-J. Wang, P.-Z. Li, Y. Chen, Q. Zhang, H. Zhang, X. X. Chan, R. Ganguly, Y. Li, J. Jiang and Y. Zhao, Sci. Rep., 2013, 3, 1149 CrossRef PubMed;
(d) B. Zheng, Z. Yang, J. Bai, Y. Li and S. Li, Chem. Commun., 2012, 48, 7025–7027 RSC;
(e) O. K. Farha, C. E. Wilmer, I. Eryazici, B. G. Hauser, P. A. Parilla, K. O'Neill, A. A. Sarjeant, S. T. Nguyen, R. Q. Snurr and J. T. Hupp, J. Am. Chem. Soc., 2012, 134, 9860–9863 CrossRef CAS PubMed;
(f) B. Li, Z. Zhang, Y. Li, K. Yao, Y. Zhu, Z. Deng, F. Yang, X. Zhou, G. Li, H. Wu, N. Nijem, Y. J. Chabal, Z. Lai, Y. Han, Z. Shi, S. Feng and J. Li, Angew. Chem., Int. Ed., 2012, 51, 1412–1415 CrossRef CAS PubMed;
(g) O. K. Farha, I. Eryazici, N. C. Jeong, B. G. Hauser, C. E. Wilmer, A. A. Sarjeant, R. Q. Snurr, S. T. Nguyen, A. Ö. Yazaydın and J. T. Hupp, J. Am. Chem. Soc., 2012, 134, 15016–15021 CrossRef CAS PubMed;
(h) Y. Yan, A. J. Blake, W. Lewis, S. A. Barnett, A. Dailly, N. R. Champness and M. Schröder, Chem. – Eur. J., 2011, 17, 11162–11170 CrossRef CAS PubMed;
(i) B. Zheng, J. Bai, J. Duan, L. Wojtas and M. J. Zaworotko, J. Am. Chem. Soc., 2011, 133, 748–751 CrossRef CAS PubMed;
(j) D. Yuan, D. Zhao and H.-C. Zhou, Inorg. Chem., 2011, 50, 10528–10530 CrossRef CAS PubMed;
(k) D. Yuan, D. Zhao, D. Sun and H.-C. Zhou, Angew. Chem., Int. Ed., 2010, 49, 5357–5361 CrossRef CAS PubMed;
(l) Y. Yan, I. Telepeni, S. Yang, X. Lin, W. Kockelmann, A. Dailly, A. J. Blake, W. Lewis, G. S. Walker, D. R. Allan, S. A. Barnett, N. R. Champness and M. Schröder, J. Am. Chem. Soc., 2010, 132, 4092–4094 CrossRef CAS PubMed;
(m) D. Zhao, D. Yuan, D. Sun and H.-C. Zhou, J. Am. Chem. Soc., 2009, 131, 9186–9188 CrossRef CAS PubMed.
-
(a) Z. Liu, L. Lv, Y. He and Y. Feng, CrystEngComm, 2017, 2795–2801 RSC;
(b) F. Chen, D. Bai, X. Wang and Y. He, Inorg. Chem. Front., 2017, 960–967 RSC;
(c) C. Song, H. Liu, J. Jiao, D. Bai, W. Zhou, T. Yildirim and Y. He, Dalton Trans., 2016, 45, 7559–7562 RSC;
(d) C. Song, Y. Ling, L. Jin, M. Zhang, D.-L. Chen and Y. He, Dalton Trans., 2016, 45, 190–197 RSC;
(e) C. Song, J. Jiao, Q. Lin, H. Liu and Y. He, Dalton Trans., 2016, 45, 4563–4569 RSC;
(f) J. Jiao, H. Liu, F. Chen, D. Bai, S. Xiong and Y. He, Inorg. Chem. Front., 2016, 3, 1411–1418 RSC;
(g) J. Jiao, H. Liu, D. Bai and Y. He, Inorg. Chem., 2016, 55, 3974–3979 CrossRef CAS PubMed;
(h) J. Jiao, L. Dou, H. Liu, F. Chen, D. Bai, Y. Feng, S. Xiong, D.-L. Chen and Y. He, Dalton Trans., 2016, 45, 13373–13382 RSC;
(i) C. Song, Y. Ling, Y. Feng, W. Zhou, T. Yildirim and Y. He, Chem. Commun., 2015, 51, 8508–8511 RSC;
(j) C. Song, J. Hu, Y. Ling, Y. Feng, R. Krishna, D.-L. Chen and Y. He, J. Mater. Chem. A, 2015, 3, 19417–19426 RSC;
(k) C. Song, J. Hu, Y. Ling, Y. Feng, D.-L. Chen and Y. He, Dalton Trans., 2015, 44, 14823–14829 RSC;
(l) C. Song, Y. He, B. Li, Y. Ling, H. Wang, Y. Feng, R. Krishna and B. Chen, Chem. Commun., 2014, 50, 12105–12108 RSC;
(m) Y. He, S. Xiang, Z. Zhang, S. Xiong, C. Wu, W. Zhou, T. Yildirim, R. Krishna and B. Chen, J. Mater. Chem. A, 2013, 1, 2543–2551 RSC;
(n) Y. He, W. Zhou, T. Yildirim and B. Chen, Energy Environ. Sci., 2013, 6, 2735–2744 RSC.
-
(a) J. Jiao, D. Jiang, F. Chen, D. Bai and Y. He, Dalton Trans., 2017, 7813–7820 RSC;
(b) Y.-T. Yan, W.-Y. Zhang, Y.-L. Wu, J. Li, Z.-P. Xi, Y.-Y. Wang and L. Hou, Dalton Trans., 2016, 45, 15473–15480 RSC.
-
(a) X. Duan, Y. Cui, Y. Yang and G. Qian, CrystEngComm, 2017, 19, 1464–1469 RSC;
(b) H.-M. Wen, G. Chang, B. Li, R.-B. Lin, T.-L. Hu, W. Zhou and B. Chen, Cryst. Growth Des., 2017, 17, 2172–2177 CrossRef CAS;
(c) L. Du, Z. Lu, L. Xu and J. Zhang, RSC Adv., 2017, 7, 21268–21272 RSC.
- M. A. E. P.-B. Mendieta, M. Negri, C. Jagusch, U. E. Hille, U. Müller-Vieira, D. Schmidt, K. Hansen and R. W. Hartmann, Bioorg. Med. Chem. Lett., 2008, 18, 267–273 CrossRef PubMed.
- D. F. Taber, T. D. Neubert and A. L. Rheingold, J. Am. Chem. Soc., 2002, 124, 12416–12417 CrossRef CAS PubMed.
-
(a) A. L. Spek, Acta Crystallogr., Sect. D: Biol. Crystallogr., 2009, 65, 148–155 CrossRef CAS PubMed;
(b) A. L. Spek, Acta Crystallogr., Sect. C: Struct. Chem., 2015, 71, 9–18 CrossRef CAS PubMed.
-
(a) Y. He, Z. Guo, S. Xiang, Z. Zhang, W. Zhou, F. R. Fronczek, S. Parkin, S. T. Hyde, M. O'Keeffe and B. Chen, Inorg. Chem., 2013, 52, 11580–11584 CrossRef CAS PubMed;
(b) Y. He, Z. Zhang, S. Xiang, H. Wu, F. R. Fronczek, W. Zhou, R. Krishna, M. O'Keeffe and B. Chen, Chem. – Eur. J., 2012, 18, 1901–1904 CrossRef CAS PubMed.
-
(a) Y. Liu, J.-R. Li, W. M. Verdegaal, T.-F. Liu and H.-C. Zhou, Chem. – Eur. J., 2013, 19, 5637–5643 CrossRef CAS PubMed;
(b) Z. Lu, L. Du, K. Tang and J. Bai, Cryst. Growth Des., 2013, 13, 2252–2255 CrossRef CAS.
-
(a) G. Feng, Y. Peng, W. Liu, F. Chang, Y. Dai and W. Huang, Inorg. Chem., 2017, 56, 2363–2366 CrossRef CAS PubMed;
(b) Z. Lu, J. Bai, C. Hang, F. Meng, W. Liu, Y. Pan and X. You, Chem. – Eur. J., 2016, 22, 6277–6285 CrossRef CAS PubMed;
(c) C.-Y. Gao, H.-R. Tian, J. Ai, L.-J. Li, S. Dang, Y.-Q. Lan and Z.-M. Sun, Chem. Commun., 2016, 52, 11147–11150 RSC;
(d) S. E. Wenzel, M. Fischer, F. Hoffmann and M. Fröba, J. Mater. Chem., 2012, 22, 10294–10302 RSC;
(e) X.-S. Wang, S. Ma, P. M. Forster, D. Yuan, J. Eckert, J. J. Lpez, B. J. Murphy, J. B. Parise and H.-C. Zhou, Angew. Chem., Int. Ed., 2008, 47, 7263–7266 CrossRef CAS PubMed.
- S. Xiang, W. Zhou, J. M. Gallegos, Y. Liu and B. Chen, J. Am. Chem. Soc., 2009, 131, 12415–12419 CrossRef CAS PubMed.
- Y. He, Z. Zhang, S. Xiang, F. R. Fronczek, R. Krishna and B. Chen, Chem. – Eur. J., 2012, 18, 613–619 CrossRef CAS PubMed.
- Z.-J. Guo, J. Yu, Y.-Z. Zhang, J. Zhang, Y. Chen, Y. Wu, L.-H. Xie and J.-R. Li, Inorg. Chem., 2017, 56, 2188–2197 CrossRef CAS PubMed.
- D. Tanaka, M. Higuchi, S. Horike, R. Matsuda, Y. Kinoshita, N. Yanai and S. Kitagawa, Chem. – Asian J., 2008, 3, 1343–1349 CrossRef CAS PubMed.
- J. W. Yoon, J. S. Lee, S. Lee, K. H. Cho, Y. K. Hwang, M. Daturi, C.-H. Jun, R. Krishna and J.-S. Chang, Chem. – Eur. J., 2015, 21, 18431–18438 CrossRef CAS PubMed.
- Y. He, R. Krishna and B. Chen, Energy Environ. Sci., 2012, 5, 9107–9120 CAS.
- K. Liu, B. Li, Y. Li, X. Li, F. Yang, G. Zeng, Y. Peng, Z. Zhang, G. Li, Z. Shi, S. Feng and D. Song, Chem. Commun., 2014, 50, 5031–5033 RSC.
- Y. Hu, S. Xiang, W. Zhang, Z. Zhang, L. Wang, J. Bai and B. Chen, Chem. Commun., 2009, 7551–7553 RSC.
- D.-M. Chen, J.-Y. Tian, C.-S. Liu, M. Chen and M. Du, Chem. – Eur. J., 2016, 22, 15035–15041 CrossRef CAS PubMed.
- F. Wang, S. Kusaka, Y. Hijikata, N. Hosono and S. Kitagawa, ACS Appl. Mater. Interfaces, 2017 DOI:10.1021/acsami.7b01568.
- Q.-G. Zhai, X. Bu, C. Mao, X. Zhao, L. Daemen, Y. Cheng, A. J. Ramirez-Cuesta and P. Feng, Nat. Commun., 2016, 7, 13645 CrossRef CAS PubMed.
- M. Zhang, B. Li, Y. Li, Q. Wang, W. Zhang, B. Chen, S. Li, Y. Pan, X. You and J. Bai, Chem. Commun., 2016, 52, 7241–7244 RSC.
- F. Moreau, I. d. Silva, N. H. A. Smail, T. L. Easun, M. Savage, H. G. W. Godfrey, S. F. Parker, P. Manuel, S. Yang and M. Schröder, Nat. Commun., 2016, 8, 14085 CrossRef PubMed.
- X. Duan, H. Wang, Z. Ji, Y. Cui, Y. Yang and G. Qian, Mater. Lett., 2017, 196, 112–114 CrossRef CAS.
- Z. Zhang, S. Xiang, Y.-S. Chen, S. M. Lee, T. Phely-Bobin and B. Chen, Inorg. Chem., 2010, 49, 8444–8448 CrossRef CAS PubMed.
- J. Li, H.-R. Fu, J. Zhang, L.-S. Zheng and J. Tao, Inorg. Chem., 2015, 54, 3093–3095 CrossRef CAS PubMed.
- Q.-R. Ding and F. Wang, Dalton Trans., 2016, 45, 7004–7007 RSC.
- Y. He, C. Song, Y. Ling, C. Wu, R. Krishna and B. Chen, APL Mater., 2014, 2, 124102 CrossRef.
Footnote |
† Electronic supplementary information (ESI) available: PXRD patterns (Fig. S1), TGA curves (Fig. S2), FTIR spectra (Fig. S3), BET and Langmuir plots (Fig. S4–S6), comparison of the pure-component isotherm data with the fitted isotherms (Fig. S7–S9), IAST selectivities for the equimolar C2H2/CH4, CO2/CH4 and C2H2/CO2 gas mixtures in ZJNU-74, ZJNU-75 and ZJNU-76 at three different temperatures (Fig. S10–S12), 1H and 13C NMR spectra (Fig. S13), Langmuir–Freundich parameters for adsorption of C2H2, CO2, and CH4 in ZJNU-74, ZJNU-75 and ZJNU-76 (Tables S1–S3), crystallographic data and structure refinement parameters (Table S4), CCDC 1558048, 1543094 and 1558537. For ESI and crystallographic data in CIF or other electronic format see DOI: 10.1039/c7qm00302a |
|
This journal is © the Partner Organisations 2017 |
Click here to see how this site uses Cookies. View our privacy policy here.