DOI:
10.1039/C7QM00305F
(Research Article)
Mater. Chem. Front., 2017,
1, 2574-2589
Electromagnetic screening in soft conducting composite-containing ferrites: the key role of size and shape anisotropy
Received
6th July 2017
, Accepted 7th September 2017
First published on 8th September 2017
Abstract
Functional nanomaterial embedded lightweight polymer composites have drawn considerable attention across wide ranges of industrial applications. In addition to telecommunication and aerospace utilities, microwave absorbing materials must possess interesting properties that ensure excellent performance, from mechanical features to functionalities. Although conducting polymer composites containing magnetic nanofillers have been utilised widely, choosing the fillers from the library of nanoparticles and their effective dispersion inside the matrix may limit their usage in terms of performance, stability and durability. In order to overcome this bottleneck, herein we explored a facile bottom-up synthetic procedure to fabricate different shapes (spherical, cubic, cluster and flower, for example) and size-controlled Fe3O4 nanoparticles, and showed the effect of shape anisotropy and size on the above properties in a model polycarbonate (PC)/polyvinylidene difluoride (PVDF) blend with multiwalled carbon nanotubes as conducting nanofillers. The superior performance in terms of microwave attenuation and mechanical properties was reported for spherically shaped Fe3O4 nanomaterials. The excellent dispersibility of small-sized nanospheres was instrumental in the improved consolidated loss tangent values, attenuation constant, and impedance matching and skin depth, synergistically resulting in a shielding efficiency of −38 dB at 18 GHz.
Introduction
High frequency millimetre waves are generally employed in various types of equipment, notably in electronic circuitry in wireless communications, and are particularly suited for the civil and military sector, including medical and aerospace applications. However, issues related to electromagnetic interference (EMI) are also proliferating at an alarming pace as an offshoot of the development of modern technologies.1,2 This has prompted experts to declare EMI as a new and prominent source of pollution. Such interference not only undermines the performance of other devices, but also creates a range of human health issues. Therefore, the desire to eliminate this unwanted electromagnetic (EM) radiation creates a mandate for the development of materials that can absorb EM for urgent use in product design.3 Metals, despite being widely consumed as a common EM-shielding material, suffer from several disadvantages such as heavy weight, low flexibility, difficult processing and susceptibility to corrosion. The search for novel and effective EM-shielding materials has triggered research in flexible and lightweight reinforced polymer-matrix-containing conducting and magnetic nanoparticles.4,5 Previously, carbon-based nanomaterials were widely used as a filler in polymer matrices due to their versatile appearance (e.g., in the form of graphene, graphene oxide, graphite, exfoliated graphite, carbon black, amorphous carbon and carbon nanotubes) and exceptional physical properties (e.g., electrical conductivity, thermal property, mechanical property and large specific surface area).6,7 Generally, conductive nanoparticles with a high dielectric constant attenuate EM radiation by reflection of the incident EM wave from the surface of the shields, which may trigger the secondary pollution. Recent studies reveal that magnetic loss is more important than conductive loss for better EM absorbing properties.8–12 However, the percolation threshold of magnetic nanoparticles is very high in the polymer matrix, which can significantly overshadow others important properties. Therefore, a combination of both conducting and magnetic nanoparticles has been proposed for reproducing synergetic behaviour in shielding efficiency (SE), where the magnetic permeability and dielectric permittivity play pivotal roles towards minimising EMI.13–16 According to the Snoek rule, ferromagnetic iron materials are ideal candidates for EMI shielding due to their high magnetic permeability and high saturation magnetisation at room temperature.17 However, high eddy current loss for the ferromagnets can be suppressed by utilising ferrite nanoparticles with a size below the ‘skin depth’, which is typically about 1 μm in the microwave frequency region.
Cao et al. reported a comparative study on ferroferric oxide/multiwalled carbon nanotube and polyaniline/ferroferric oxide/multiwalled carbon nanotube (MWCNT) multiheterostructures for highly effective microwave absorption phenomena, where spherical shape Fe3O4 nanoparticles (10–20 nm) were used.18 They claimed that due to their high complex permittivity and permeability, Fe3O4/MWCNTs are highly effective in shielding electromagnetic radiation. Singh et al. suggested a high-performance Fe3O4 (15 nm) infiltrated rGO–MWCNT forest sandwich network for suppressing electromagnetic pollution.19 Hekmatara et al. reported the synthetic procedure of SiO2-coated Fe3O4–MWCNT nanocomposites for EM absorption application, where the size of Fe3O4 nanoparticles was 7–18 nm.20 Liu et al. developed nanocomposites based on an epoxy matrix with nano-sized MWCNTs, Fe3O4 and Fe fillers for EM-shielding applications.21 They showed that the dielectric permittivity and the magnetic permeability of nano-Fe3O4, nano-Fe and CNT composites increase with increasing mass fraction, and that the EMI shielding property of the three types of composite are all dominant over dielectric loss, rather than magnetic loss. Bhattacharya et al. suggested TiO2 coating on MWCNT/Fe3O4 composites help in the microwave absorption phenomena.22 Wang et al. showed the synthetic route of growing Fe3O4 nanocrystals with a core size of 8 nm on MWCNT in high density, which exhibits high-performance EM absorption phenomena in the 2–18 GHz frequency range.23 Lu et al. showed a synthetic route for the assembly of grape-like ferroferric oxide and MWCNTs, where Fe3O4 nanocrystals are spherical in shape and 6–16 nm in size.24 Hekmatara et al. suggested that microwave attenuation efficiency was also influenced by alignment of MWCNTs to the electric field vector of incident EM waves in MWCNT/Fe3O4 composites.25 They synthesised MWCNT/Fe3O4 composites with varying loading concentration of Fe3O4 nanoparticles in the composites in which the average size of Fe3O4 cluster was in the 7–18 nm region. Liu et al. showed a solution-induced synthetic procedure of MWCNT/Fe3O4 composites for EM absorption phenomena where the size of the spherical-shaped Fe3O4 nanoparticle was 150 nm.26 The EM wave absorbing property of silicone rubber filled with carbonyl iron particles (CIPs) and multiwalled carbon nanotubes was examined by Xu et al., and the diameter of the flaky CIPs was found to be in the region of 5 μm.27 A chemoselective route to induce Fe3O4@ZnO core–shell nanoparticle-decorated carbon nanotubes to form MWCNT/Fe3O4@ZnO heterotrimers has been developed by Wang et al., in which the size of the Fe3O4 nanoparticles was in the 8–20 nm region.28 The significant interfacial polarisation and synergetic interaction generated between dielectric and magnetic absorbers results in the formation of MWCNT/Fe3O4@ZnO heterotrimers with high-performance microwave absorption in the entire X band. Tsonos et al. compared the effect of MWCNT/Fe3O4 and graphene/Fe3O4 nanoparticles in a PVDF matrix in which the size of the Fe3O4 nanoparticles was in the nm region.29 The have spuriously shown MWCNT/Fe3O4 nanoparticles in 3–10 GHz frequency region due to corresponding loss parameters. Wu et al. showed the effect of elliptical core–shell nanorings of Fe3O4/C for efficient low frequency microwave absorption.30 Guo et al. suggested that polypyrrole coated Fe3O4 also enhanced the EM wave absorption due to their enlarged surface area, eddy current loss and anisotropic energy.31 Huang et al. showed Fe3O4 nanofibers have EM absorption properties due to their interfacial polarisation and magnetic loss.32 Li et al. suggested the compact coating of Fe3O4 nanoparticles on MWCNTs also efficient for EM-shielding efficiency.33 Cui-Ling et al. prepared MWCNT/Fe3O4 hybrids using a hydrothermal treatment method for EM-shielding application.34 Cube-shaped (15–20 nm) Fe3O4 nanoparticles were attached on MWCNTs by this method and resulted in −18.2 dB shielding effectiveness at 12.05 GHz frequency.
Interestingly, Fe3O4 nanoparticles with different morphologies can exhibit exciting behaviour due to their distinct properties associated with various size and shape anisotropies. Generally, Fe3O4 possesses an inverse spinel structure in which the tetrahedral sites are occupied by Fe(III) and the octahedral sites are occupied by Fe(II) and Fe(III), with a space grouping of fd3m. The unit cell of Fe3O4 contains 32 O2− ions, which accounts for the cubic close-pack structure along with the [111] direction, and the crystals are overlaid with octahedral and mixed octahedral/tetrahedral layers along with this direction. The high-index planes usually have a higher surface energy. The sequence of γ(111)< γ(100) < γ(110)< γ(220) for the face-centred-cubic phase can be generated from the distance between those planes and the central Wulff's point. As a result, the Fe3O4 nanoparticles are surrounded mostly by {111}, and exhibit an octahedral morphology. However, the crystal shape is defined by the ratio (R) of growth rate in the 〈100〉 direction to that in the 〈111〉 direction. The shortfall of most studies to date involves particle size distribution on the matrix due to chemical incompatibility. It has been shown in the literature that the characteristic length and surface-to-volume ratio of nanoparticles and their distribution with effective volume fraction in the matrix will significantly influence the dielectric and magnetic properties of the final structure. In particular, saturation magnetisation, which is one of the main reasons behind the EM absorption, depends on the particle's shape anisotropy and size. However, above the critical size, distribution of nanoparticles in the matrix is another comprehensive parameter for attaining other properties.
Despite the success of several studies in the development of soft EM absorbing materials, here we exclusively concentrate on several precise factors which can clearly demonstrate the effect of optimum Fe3O4 nanoparticle size in terms of shielding responses of an immiscible polymer-matrix-containing conducting additives. We have employed well discussed wet chemical methodologies for synthesising Fe3O4 nanoparticles with wide ranges of size and shape anisotropies by careful tuning of the reaction conditions. Herein, we also studied the effect of incorporation of shape- and size-controlled Fe3O4 nanomaterials into model PC/PVDF blend with conducting inclusion in terms of morphology, mechanical properties, rheological properties and AC electrical conductivity. Moreover, spherical-shaped Fe3O4 nanoparticles showed superior shielding while blended with conducting MWCNTs due to better dispersion, which eventually enhanced the conduction loss and interfacial polarisation. Furthermore, loss tangent values, attenuation constant, impedance matching and skin depth analyses evidently proved that the highest SE was obtained through synergistic inclusion of spherical-shaped Fe3O4 and MWCNTs. However, saturation magnetisation and magnetic permeability played a pivotal role when both NS1 and NS2 possessed comparable dispersibility. We have also deduced a correlation between saturation magnetisation and synthesised ferrite nanoparticles with size and shape anisotropy. This further correlates with the SE of other nanocube (NCu), nanocluster (NC) and nanoflower (NF) types of ferrites.
Experimental section
Materials
PVDF (Kynar-761, with a Mw of 440
000 g mol−1) was kindly provided by Arkema. Polycarbonate (Lexan-143R) was obtained from Sabic (MFI 11 g/10 min). Pristine MWCNTs (length, 1.5 μm and diameter, 9.5 nm) were procured from Nanocyl SA (Belgium). Iron(III) acetylacetonate, Jeffamine ED-600 and 4-dodecylbenzenesulfonic acid (DBSA) were procured from Sigma-Aldrich. Analytical grades of chloroform, ethanol, N,N-dimethylformamide, ethylene glycol, HNO3, sodium thiosulfate and tetrahydrofuran were obtained from commercial sources.
Synthesis of sphere-shaped Fe3O4 nanoparticles (NS1, diameter 15 ± 5 nm)
Here we have synthesised sphere-shaped Fe3O4 nanoparticles by thermal decomposition of iron(III) acetylacetonate in tetraethylene glycol medium. Initially 1 mmol of iron(III) acetylacetonate was dissolved in 50 ml tetraethylene glycol with vigorous stirring and sonication. Then, the mixture was heated at 240 °C for 3 h. Finally, a black residue was obtained and washed several times with water/ethanol mixture.
Synthesis of sphere-shaped Fe3O4 nanoparticles (NS2, diameter 25 ± 6 nm)
Spherical Fe3O4 nanoparticles with an average diameter of 25 nm were synthesised via coprecipitation of Fe2+ and Fe3+. Initially, FeSO4·7H2O (1 mmol) and FeCl3 (2 mmol) were dissolved in 40 ml of ethylene glycol with vigorous stirring at room temperature. Later, 4 ml of 25% ammonia solution was added into the reaction mixture and stirring was continued for another 20 min. The mixture was then transferred to a Teflon-lined autoclave and the mixture was maintained at 210 °C for 3 h. Finally, the mixture was cooled to room temperature and the resulting particles were collected by magnetic decantation followed by repeated washing with water/ethanol mixture, and finally dried at 60 °C.
Synthesis of Fe3O4 nanocubes (NCu, diameter 100 ± 10 nm)
Cube-shaped nanoparticles were obtained by the hydrothermal reduction of FeCl3 in tetraethylene glycol medium in the presence of hydrazine hydrate solution. Initially, FeCl3 was dissolved in tetraethylene glycol and sonicated for 30 minutes, following the drop-wise addition of 2 ml of hydrazine hydrate. Then, the solution was placed in a Teflon-lined autoclave and the whole reaction mixture was maintained at 210 °C for 24 h. Finally, a black residue was isolated and filtered using an ethanol/water mixture several times, and vacuum dried at 60 °C.
Synthesis of nanoclusters of Fe3O4 nanoparticles (NC, diameter 75 ± 10 nm)
Initially, iron(III) acetylacetonate was dissolved in ethylene glycol, and the solution was placed in a Teflon-lined autoclave. NaOH was added to maintain the pH at 9–10 and the solution was maintained at 210 °C for 24 h. Finally, a black residue was isolated and washed several times with toluene, and vacuum dried at 60 °C.
Synthesis of flower-shaped Fe3O4 nanoparticles (NF, diameter 150 ± 15 nm)
The iron(III) acetylacetonate was sonicated to dissolve in ethylene glycol, and the solution was placed in a Teflon-lined autoclave. NaOH was added to maintain the pH at 9–10, and Jeffamine ED 600 polyetheramine was added. Then the mixture was kept at 210 °C for 24 h. Finally, a black residue was isolated and washed several times with toluene, and vacuum dried at 60 °C.
Preparation of blends
All blends with different nanoparticles were prepared by using a Hake minilab-II melt compounder under a nitrogen atmosphere at 260 °C with a rotational speed of 60 rpm for 20 min. MWCNT concentration was kept constant for all the blend compositions; i.e. 3 wt%. In addition to MWCNTs, 3 wt% of Fe3O4 nanoparticles was also present when the blend contained both conducting and magnetic nanoparticles. It is important to note that only 3 wt% Fe3O4 nanoparticles containing blends did not showed no shielding effectiveness. For this reason, we are only interested here in both nanomaterials together in a blend, and report their effect in the subsequent experiments.
Characterisation
Transmission electron micrographs (TEM) images were procured utilising an FEI Technai F20 instrument operating at an accelerated voltage of 200 kV. A Sirion XL30 FEG scanning electron microscope (SEM) with an accelerated voltage of 10 kV was utilised to determine the morphology of the PC/PVDF blends and synthesised nanoparticles. The magnetic properties of the synthesised nanoparticles were assessed using a Lakeshore Vibratory Sample Magnetometer (VSM) with an applied force of −8000 to 8000 Oe at room temperature. X-ray diffraction (XRD) was recorded utilising an XPERT Pro from PANalytical. A Cu-Kα radiation source (l = 1.5406 Å, 40 kV and 30 mA) was used to decipher the XRD profile of different nanoparticles. A calibrated differential scanning calorimeter (DSC; TA Instrument) was used for DSC experiments, where the sample heating rate was 10 K min−1. A room-temperature universal testing machine (UTM) was used for evaluating the mechanical properties of the various blend structures. A 5 mm min−1 cross-head speed was used for all experiments. Prior to measurement, dumbbell-shaped samples were prepared by compression moulding. A discover hybrid rheometer (DHR 3, TA Instrument) was used to study the melt rheology of the various blends. A 25 mm diameter parallel-plate geometry was used for these measurements. Room-temperature electrical conductivity of the blend was contemplated utilising an Alpha-N Analyser, Novocontrol (Germany) in a frequency range from 0.1 Hz to 10 MHz. EM interference shielding was measured by utilising an Anritsu MS4642A Vector Network Analyser (VNA). A Damaskos MT-07 coaxial connector was utilised to measure the SE.
Result and discussion
Characterisation of shape- and size-controlled Fe3O4 nanoparticles
Nanofabrication of magnetite particles with variable size and shape anisotropy most commonly involves thermal decomposition of an iron precursor.35 The first method utilises a high-boiling solvent in presence of organic surfactants/capping agents, resulting in uniform monodisperse magnetite particles with a hydrophobic shell.36 The polyol method, on the other hand, is another viable alternative for the synthesis of magnetite nanostructures, in which the iron precursor, usually iron(II) acetylacetonate, is thermally decomposed in a refluxing polyol solvent.37 This method also rules out the use of a surfactant/capping agent, as polyol itself acts as a reducing agent and stabiliser to control the particle growth and prevent inter-particle aggregating, thereby producing monodisperse nanoparticles.38 The advantages of this approach are the possibility to kinetically control the experimental conditions and the ease of scale-up.38
The mechanism of growth of nanoparticles from the solution phase thermal decomposition method can be illustrated by the LaMer model of nucleation, which involves the formation of a metal complex by the dissolution of metal precursor in an organic solvent with a high-boiling temperature and usually in the presence of surfactant molecules.39 At a certain temperature, the metal complex becomes unstable and decomposes into intermediate species or seeds which act as building blocks for the nanoparticles. The concentration of the intermediate species increases until reaching a high level of supersaturation at which a new phase, nuclei, is formed spontaneously consuming the intermediate species; this process is known as homogeneous nucleation.40 The nucleation reduces the concentration of the monomers until no further nucleation proceeds. The remaining monomers precipitate on the surface of the nuclei, and the primary particles increase their dimensions continuously; this stage is known as the growth process. Typically, the nanoparticles formed acquire uniform morphological characteristics, since nucleation and the growth process are entirely separate steps in time due to the fact that the nucleation rate is sufficiently high.41
XRD diffractograms of the various magnetite nanostructures are shown in Fig. 1. The corresponding peaks were identified and indexed in position and relative intensity to the characteristic pattern corresponding to the inverse spinel structure of magnetite Fe3O4 (JCPDS International Centre for Diffraction card no. 33-0664). The presence of all major inverse spinel peaks for the nanostructures indicates that magnetite is the main phase in the samples.42 The crystallites sizes, Dxrd, of the nanostructures were calculated using Scherrer's equation,43 based on the (311) peak: Dxrd = 0.9λ/β
cos
θ where λ is the X-ray wave length, θ is the Bragg's angle and β is the full width at half of the maximum intensity (FWHM). Dxrd values obtained lie in the range 15–55 nm, thus corroborating scanning electron micrograph (SEM)/TEM observations. For the nanospheres (NS1, NS2), the average crystallite sizes were calculated to be close to TEM values, being 14 nm for the NS1 and 24 nm for the NS2. However, for the NCs and NFs, the Dxrd proved to be inefficient, as the final nanostructures formed following a hierarchical addition of nanoscale building blocks. Furthermore, presence of sharper and narrower peaks highlighted the fact that greater crystallinity was achieved in the NF. For smaller-sized nanostructures, a distortion in the arrangement of the surface atoms could lead to broadening and defects in the diffraction patterns.44Fig. 2A–E shows the SEM of different nanostructures of Fe3O4 samples. The morphology of the particles is observed to be directly dependent on the types of surfactant/polymeric capping agents, and solvent-cum-reducing agents employed and the reduction methodologies. The SEM images of nanosphere (NS1 and NS2) surface morphology consist of a nearly spherical structure. On the other hand, the images of NFs and NCs illustrate the hierarchical aggregates of nanoscale building blocks coalesced to form clusters and flower-like morphologies of a nearly monodispersed nature. This reflects the role of the triblock copolymer as a capping agent in controlling the growth rate of various faces of the Fe3O4 crystal towards the formation of the NF. The role of the triblock copolymer in the growth and exfoliation of nano- and microflowers has been investigated previously.45 The evolution of the final morphology results from the nuclei generated and subsequent formation seed in a kinetically controlled process. The formation of seed from clusters indicates a critical stage of nanoparticle synthesis, beyond which structural fluctuation becomes inevitable so that the seeds then undergo a diffusion–dissolution mechanism.40 Following this, diffused atoms on the surface of the newly formed nanoparticles orient themselves in a preferential crystal growth direction. Hydrophilic parts of the triblock copolymer are preferentially adsorbed onto the nanoparticle surface to form uniform nanospheres. With increasing reaction time, decomposition of the precursor slowed down due to Oswald ripening. This results in the hierarchical aggregation of nanoparticles towards the formation of various aggregated morphologies, including NCs and NFs. The SEM of NCs shows a distinct cuboidal shape with a high degree of monodispersity. The average size of the NCs is observed to be 100 nm.
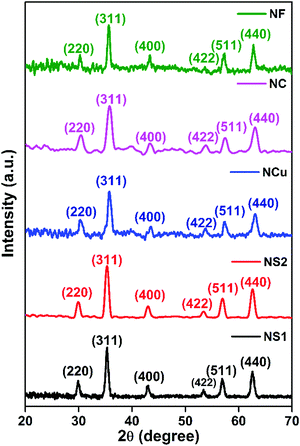 |
| Fig. 1 XRD plot of various shape-controlled FeO4 nanoparticles. | |
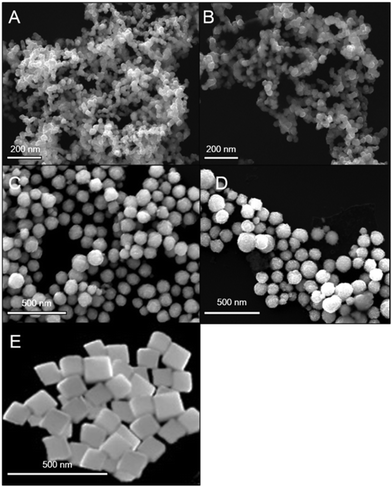 |
| Fig. 2 SEM micrograph of (A) NS1, (B) NS2, (C) NC, (D) NCF and (E) NCu. | |
Conventional TEM imaging revealed the effect of precursor, solvent and surfactant/polymeric capping agent on the morphology, shape and size distribution of the different nanostructures of Fe3O4 (Fig. 3). The TEM images of NS1 and NS2 samples (Fig. 3A and B) show that the Fe3O4 nanoparticles are largely non-agglomerated, roughly monodisperse spheres with average diameters of 15 ± 5 nm and 25 ± 6 nm, respectively. This observation approximately echoes the average crystallite size of the nanocrystals calculated from X-ray diffractograms. The spherical shapes evolve during the growth of nanoparticles from a precursor solution as the nucleation rate per unit area is isotopic at the interface between the Fe3O4 magnetic nanoparticles. To minimise the surface free energy, the growth rate during structural evolution is uniform along different directions. The sphere has the smallest surface area per unit volume of any shape; therefore, nanoparticles largely appear to be spherical in these cases. The TEM images for the NC and NF (Fig. 3C and D) prepared in presence of polyol media and triblock copolymer as a capping agent were found to be hierarchically grown, and are shown in Fig. 3. Fig. 3C and D further shows that NC and NF have an average diameter of 75 ± 10 nm and 150 ± 15 nm, respectively. The NCs (Fig. 3G) are nearly monodisperse in nature, and the average size was calculated to be 100 ± 10 nm. On the other hand, high-resolution transmission electron microscopy (HRTEM) (3E, 3H) provided a further insight into the structural features of the Fe3O4 NC and NF. The structural analysis of the 2D-FFT patterns of the NS2 nanospheres (Fig. 3F) indicated the presence of the magnetite phase exclusively in the sample. The HRTEM of NS1 and the NF indicated the possible growth direction, while the presence of lattice fringe with a periodic spacing of 0.25 nm (corresponding to the 311 plane) illustrate the nanocrystalline nature of the NS1.
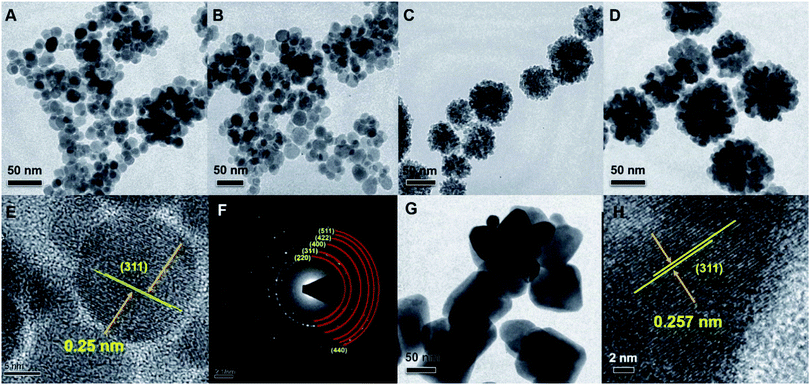 |
| Fig. 3 TEM image of (A) NS1, (B) NS2, (C) NC, (D) NF and (G) NCu. HRTEM images of corresponding NS1 and NF are shown in (E) and (H), respectively. Selected area electron diffraction (SAED) of NS2 is shown in (F). | |
Magnetic hysteresis (M–H) loops of all the Fe3O4 nanostructures were investigated using a vibrating sample magnetometer at room temperature (300 K), and the data are presented in Fig. 4A and B. While comparing the effect of surfactant, size and shape anisotropy on the magnetic properties of the different magnetite particles, we found interesting variations in terms of saturation magnetisation, coercivity and remanence. Saturation magnetisation for highly anisotropic NCu was observed to be 75 emu g−1 with strongly ferromagnetic behaviour. The Ms for NCu was slightly lower than that of the bulk magnetite value (92 emu g−1).46 The saturation magnetisation of nanoparticles is usually lower than that of the corresponding bulk sample, and decreased with reduction of the particle size as observed in the other magnetite specimens in this study.44 The Ms for NS1, NS2, NC and NF were measured as 58 emu g−1, 62 emu g−1, 65 emu g−1 and 68 emu g−1, respectively. The trend clearly illustrates the size-dependent magnetisation behaviour in the Fe3O4 samples. A similar analogy can also be extended for the NFs and NCs, as the former with a higher average size showed a higher saturation magnetisation.47 All samples demonstrated room-temperature ferromagnetic behaviour, except the nanocrystalline NS1 which is a superparamagnet with negligible coercivity and remanence. The highest coercivity was reported for NF (163 Oe), followed by NCu (132 Oe). The effect of size and anisotropy factor both combined for NF to reproduce a significant coercivity value. Therefore, it is reasonable to suggest that the growth along the easy magnetisation axis for bigger nanoparticles should improve the magnetic properties. During the measurement, the Fe3O4 NCs tend to align along the line of magnetic force. Magnetisation orientates all the net magnetic moments of the Fe3O4 NCs along the line of magnetic force. As a result, the saturation magnetisation (Ms) could be significantly improved. The presence of magnetic dead layers (MDL) on the particle surface could also contribute negatively towards the net magnetic saturation.48 The MDL thickness can be estimated from eqn (1):49
| 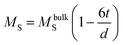 | (1) |
where
MbulkS is the bulk saturation magnetisation of Fe
3O
4,
d is the diameter of the particle and
t is MDL thickness. The existence of MDL on the surface might be caused by several factors, including surface spin canting
50 or loss of long-range order in surface layers.
51 Putting in the values of other the parameters in
eqn (1), we evaluated the MDL thickness for the samples. It can be seen that small fluctuations in
Ms values and the larger size of the nanoparticles resulted in a significant rise in ‘
t’ for NF (6.52 nm); whereas for NCu and NC, it was almost similar (3.66 nm and 3.6 nm, respectively) (
Table 1). These all point to the effect of MDL, which clearly has a significant effect on the magnetic properties of the Fe
3O
4 nanostructures.
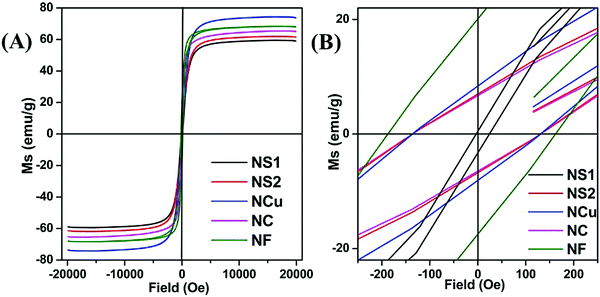 |
| Fig. 4 (A and B) VSM, magnetisation plot of synthesised nanoparticles. | |
Table 1 Magnetisation data obtained from VSM plot
Sample name |
M
s
|
M
r
|
H
c
|
Size (d/nm) |
t (nm) |
NS1 |
58 |
— |
26 |
15 ± 5 |
1.07 |
NS2 |
62 |
7.3 |
129 |
25 ± 6 |
1.6 |
NC |
65 |
6.5 |
130 |
75 ± 10 |
3.66 |
NCu |
68 |
8.3 |
131 |
100 ± 10 |
3.07 |
NF |
75 |
20 |
163 |
150 ± 15 |
6.52 |
Blend morphology and selective localisation
Monitoring the phase morphology is crucial as it decides the final properties of the blend structures. In general the lack of specific interaction between the components leads to heterogeneous morphology.15,52 A co-continuous type of morphology is observed for control 50/50 PC/PVDF blend where the PC phase was selectively etched out from the cryofractured sample to improve the contrast between the phases (Fig. 5A–F). Herein, we have used the PC/PVDF blend as a model blend due to its wide window in terms of composition, resulting in co-continuous morphology and the polarity-induced dispersion ability of filler nanomaterials which we have obtained in our previous studies.8,9 With inputs from SEM, we can ascertain the fact that with the addition of different filler nanoparticles, overall co-continuous morphology was retained. However, adding the greater size of Fe3O4 nanoparticles, the overall morphology was observed to coarsen slightly as the dispersion of filler nanoparticles inside the matrix was unimpressive due to substantial entropic penalty.
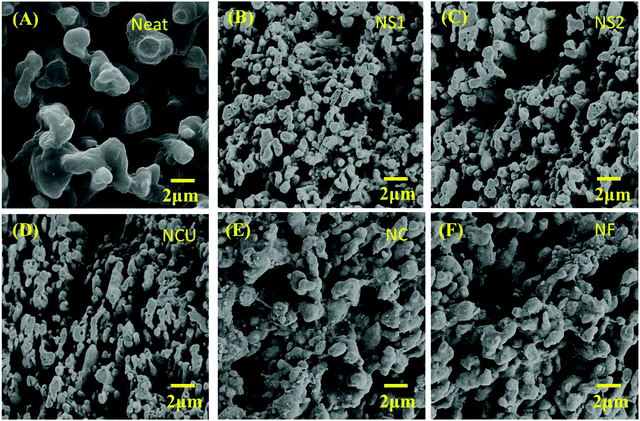 |
| Fig. 5 SEM micrograph of (A) neat PC/PVDF, (B) NS1, (C) NS2, (D) NCu, (E) NC and (F) NF. | |
Our previous studies indicate that all the nanoparticles were selectively localised in PVDF to lower the polarity mismatch among the components.8,53 As is the case here, high magnification SEM micrograph of cryofractured showed extruded strands where PC was etched out using chloroform (inset of Fig. 6A). This echoes our previous observations, in that all the nanoparticles are localised in PVDF.8,47 Furthermore, the EDAX spectra (Fig. 6A) of cryofractured (PC etched) samples containing different nanoparticles (here NS2 and MWCNT) confirmed the selective localisation in PVDF. Furthermore, by selective dissolution experiments, we can ascertain the selective localisation of nanoparticles in the co-continuous PC/PVDF blend. As shown in Fig. 6B, after the extraction of PC for 72 h in chloroform, the obtained solution is colourless; whereas, a black-coloured solution was obtained after the extraction of PVDF by dimethylformamide (DMF). This observation clearly indicates that all the nanoparticles were selectively localised in the PVDF phase.
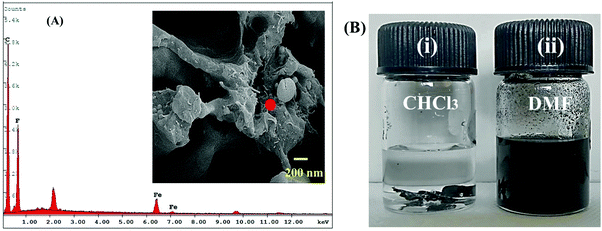 |
| Fig. 6 (A) EDAX spectra of NS2 containing blend (inset shows high magnification SEM micrograph of NS2 containing blend), (B) solution dissolution test of NS2 containing sample (i) samples were dipped in chloroform to etch out the PC phase, a clear solution indicates no migration of nanoparticles in PC and (ii) samples were dipped in DMF to etch out PVDF phase, a black colour solution indicated nanoparticles were selectively localised in PVDF. | |
Blend rheology and crystallisation
The flow characteristics of blends were assessed by melt rheology, which is an important tool to detect the processability of the composites.54,55 However, due to the immiscibility between the components of the blends, the characteristic flow behaviour is complex as the viscoelastic properties and obtained microstructures during blending are interdependent.56 The state of dispersion of the nanoparticles can also be comprehended from the viscoelastic properties of the blend. Fig. 7A shows the complex viscosity of the constituents and various PC/PVDF blends at 260 °C. It is observed that all the investigated components exhibit strong shear thinning behaviour at a lower frequency except PC, which showed almost Newtonian behaviour within the measured frequency range. It has also been observed that the addition of filler nanoparticles into the blend results in a significant increment of complex viscosity. Moreover, the enhancement is more pronounced in the lower frequency region where sufficient time was offered for chain relaxation. Such an observation clearly suggests that filler nanoparticles constrain the macromolecular motion in a given flow field. An extensive enhancement in complex viscosity is also observed after addition of Fe3O4 nanoparticles along with the MWCNTs into the blend. However, interestingly, it is worth mentioning that the complex viscosity finally scales up with the shape of the Fe3O4 nanoparticles. Generally, the dispersion of filler nanoparticles plays a vital role. The smaller size spherical-shaped Fe3O4 nanoparticles (NS1 and NS2) are dispersed easily in the matrix and they hinder the macromolecular motion to a greater extent, which steeply enhances the overall complex viscosity. However, due to the larger sizes, NCu, NC and NF nanostructures are not dispersed well and they form agglomerates inside the matrix which is also evident from the coarsened SEM micrograph. Localisation of the filler nanoparticles and their dispersion in the matrix was further assessed by the DSC crystallisation study. In general, the crystallisation temperature is greatly affected by the presence of various rigid nanoparticles and their distribution inside the matrix. As PVDF is a semicrystalline polymer, this study is important. It is observed that the crystallisation temperature of PVDF is greatly influenced following the addition of various nanoparticles, which clearly indicates that all the nanoparticles are preferentially localised in PVDF. The crystallisation temperature shifted to 138 °C from 134 °C (neat PC/PVDF) when 3 wt% MWCNTs were incorporated into the blend (Fig. 7B). This clearly suggests that MWCNTs serve the role of a nucleating agent for PVDF, thus accelerating the overall process. Further addition of Fe3O4 nanoparticles introduces further sites of crystallisation, thereby hastening the process even further. The crystallisation temperature shifted to 141 °C when NS1 and NS2 were incorporated, but the shift was slightly lower when NCu, NC and NF were incorporated into the blend, which clearly demonstrates the different degrees of dispersibility from different shape- and size-controlled Fe3O4 nanostructures in the blend.
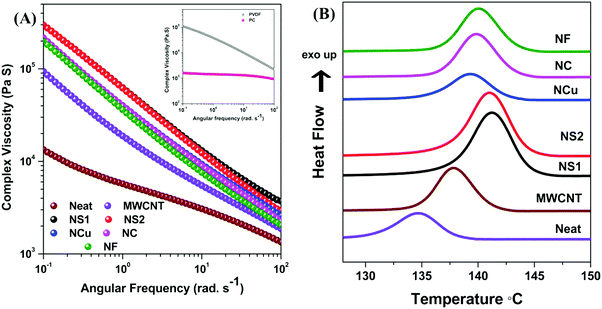 |
| Fig. 7 (A) Complex viscosity plot of various blends and (B) DSC crystallisation plot of various blends. | |
Mechanical properties
Mechanical properties of a multicomponent system are a crucial parameter and rely exclusively on the final morphology of the blend structure and interfacial interaction with blend components.57,58 Therefore, the coarse morphology inevitably leads to catastrophic failure due to poor stress transfer at the interfacial region. However, the structural properties post addition of filler nanoparticles are directly related to the obtained hierarchal microstructures.59 Several microstructure parameters, such as matrix property, localisation of filler nanoparticles, percolation threshold and interfacial bonding play pivotal role towards determining the final mechanical properties of the composites.59 The addition of MWCNTs into the blend efficiently increased the Young's modulus by ca. 38% (Fig. 8A) and ultimate tensile strength by ca. 20% (Fig. 8B). This can be explained by the fact that polymer chains are absorbed onto the MWCNTs surface and offer greater restriction against deformation. However, interestingly, elongation at break is greatly sacrificed due to the poor interfacial adhesion between the polymer chain and MWCNTs (Fig. 8C). Further addition of nanoparticles into the blends deteriorates the overall mechanical properties of the blend. However, we observed that the mechanical properties are also scaled up with the quality of dispersion of Fe3O4 nanoparticles in the PVDF matrix. As smaller-sized particles are easily dispersed in the PVDF matrix along with MWCNTs, a slightly improved Young modulus and ultimate tensile strength is shown compared with that of larger-sized particles.
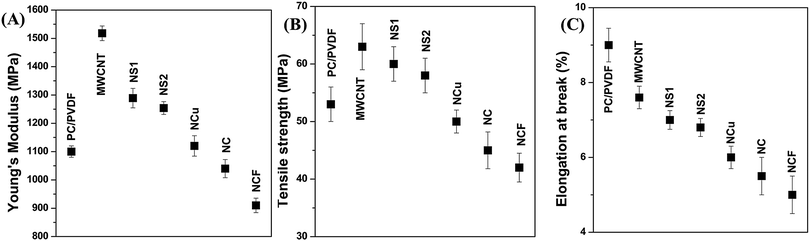 |
| Fig. 8 Mechanical properties of various blends (a) Young's modulus, (b) tensile strength, (c) elongation at break. | |
Electromagnetic shielding effectiveness
The SE of a material is defined as the ability to attenuate the incident EM radiation, and is expressed in terms of dB. Attenuation ability is measured by the ratio of incident power (Pi) and transmitted power (Pt) of the incoming radiation. In general, when the incoming radiation interacts with any shield material, the incident power can be divided into three sections; reflected power, absorbed power and transmitted power. Therefore, higher values in EM attenuation signify a lower amount of energy passing through the shield, where most of the radiation is either reflected or absorbed by the shield. The reflection power may be generated by surface reflection (SER) from the free charge carriers into the system and by multiple reflections (SEMR) from the interconnected conducting layers.60 Further, shielding by absorption (SEA) is generated by the dielectric and magnetic loss parameters. Therefore, the total SE mainly depends on these three parameters and can be expressed as a combination of these three parameters (eqn (2)).2 | SET = SER + SEMR + SEA = 10 log(Pt/Pi) | (2) |
However, due to increased absorption of reflected waves from the internal surface of the shield, multiple reflections can be ignored when the shield thickness is greater than the skin depth of the material. In this context, total shielding efficiency (SET) can be expressed as SET = SER + SEMR. From the VNA, we evaluate SET by using scattering parameters. The S-parameters S11 (or S22) and S12 (or S21), respectively, which can be correlated with the reflectance R and transmittance T are given by these equations: R = |S11|2 = |S22|2; T = |S12|2 = |S21|2; giving absorbance as A = (1 − R − T). Experimental reflection and absorption losses can be expressed as SER = −10
log10(1 − R) and SEA = −10
log10[T/(1 − R)].2,61 However, the commercially fixed standard for typical EMI shielding materials corresponds to shielding effectiveness value of −20 dB, which is equivalent to an attenuation of 99% of the total incident energy. Fig. 9A depicts a frequency-dependent SET of various designed blend structures. It is clearly observed that the blend containing 3 wt% MWCNTs shows a maximum –22 dB of total shielding effectiveness at 18 GHz frequency, while the neat blend is transparent to EM radiation. Further addition of Fe3O4 nanoparticles into the blend significantly enhances the SE. However, interestingly, the enhancement is scaled up with different shapes and sizes of the Fe3O4 nanoparticles. It is obvious that when the EM wave propagates through thick shield material, it results in attenuation of incident radiation by reflection or absorption, with negligible transmission. The magnitude of shielding by reflection depends on the surface conductivity and can be estimated theoretically by this equation:
; where (σT) corresponds to the total conductivity of the shield and (μr) represents the relative permeability of the shield. Shielding by absorption can also be estimated theoretically by this equation:
.4
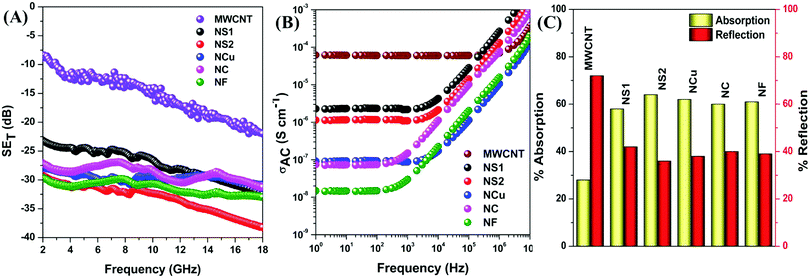 |
| Fig. 9 (A) Total shielding effectiveness, (B) AC electrical conductivity, (C) percent absorption reflection of various blends. | |
The reflection is mainly regarded as a primary mechanism of shielding when the blend possesses a sufficient electrical conductivity for carrying the charge.1 Evidently, an interconnected network of conducting filler nanoparticles inside the insulating blend matrix is crucial.62–64 Hence, selective localisation, which is the driving force to enhance the effective filler concentration, results in improved conductivity of the overall blend structure. The high magnification SEM micrograph, EDS experiment, selective dissolution test and DSC crystallisation study revealed that all the filler nanoparticles are selectively localised in PVDF, as shown previously. A very high AC electrical conductivity is observed from Fig. 9B, when only MWCNTs were incorporated as filler nanoparticles in the blend. The observed change in conductivity can be attributed to the formation of an interconnected conducting network inside PVDF, which readily enhances the SE maximum from 0 to –22 dB at 18 GHz frequency. However, after addition of Fe3O4 nanoparticles, a dramatic change in AC electrical conductivity data is observed, which is possibly attributed to the insulating property of Fe3O4 nanoparticles along with the deformation of the conducting network formed by MWCNTs. As the insulating property manifests similarly whenever a fixed amount of Fe3O4 nanoparticles were incorporated into the blend (below percolation threshold), the deformation of conducting network is a deciding factor. Due to the smaller size and relatively good dispersion, NS1 and NS2 are more efficient in retaining the conducting network structure of MWCNTs within the matrix. In contrast, agglomeration and a larger size impedes the conducting network more in the case of NCu, NC and NF nanoparticles, thus implying their effect on the magnitude of AC electrical conductivity. However, the SE has increased significantly after addition of Fe3O4 nanoparticles. The decrease in conductivity may enhance the impedance matching phenomenon, which guides the incident EM waves through the shield material instead of surface reflection. However, the presence of magnetic nanoparticles inside the shield material, which largely accounts for the enhancement of the magnetic permeability of the blend, is responsible for the shielding by absorption due to the interaction with incoming radiation. However, interestingly, although the same quantity of Fe3O4 nanoparticles was added into the blends, the SE is not the same for all Fe3O4 nanoparticles, which clearly suggests some other governing parameters here. From the equation of shielding by absorption, it is clear that absorption of incident EM radiation depends mainly on the high magnetic permeability values, whereas the high initial permeability depends on the saturation magnetisation value according to this equation: μi= Ms2/(aKHcMs + nλ), where material compositions are determined by the constant a and b, λ is the magnetorestrictive constant, ξ is the elastic strain parameter of the crystal, K is the proportionality coefficient, Ms is the saturation magnetisation and Hc is the coercivity.65,66 We observed that although the saturation magnetisation is maximum for NCu particles, the SET is observed to be highest for NS2 particles. It is likely that this observation will lead to a more detailed characterisation of blends to help find the actual reason behind the elevation of absorption-driven SE (Fig. 9C).
Mechanism of shielding
The shielding mechanism depends on the relative permeability and permittivity values. Therefore, in order to understand the proper shielding mechanism, the complex (relative) permittivity (εr) and the complex relative permeability (μr) of materials can be measured by the well-established line theory. In an air field coaxial line, the reflection coefficient can be expressed as (eqn (3)):9 | 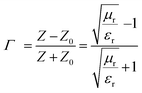 | (3) |
When the thickness of the specimen is t, then transmission coefficient can be expressed as (eqn (4)): | 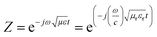 | (4) |
The characteristic impedance of the specimen mounting region is Z, whereas Z0 is the characteristic impedance through the coaxial line. Now, the S-parameters obtained from VNA can be used to calculate the reflection coefficient according to the following equation,
where,
and V1 = S21 + S11; V2 = S21 − S11. Further, | 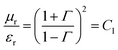 | (5) |
| 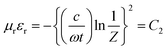 | (6) |
Therefore, the complex permittivity can be expressed as
and the complex permeability as
. These complex permittivity and permeability parameters are frequency dependent. The real part corresponds to storage ability due to polarisation of charges, whereas the imaginary part represents the dissipated energy which is associated with the loss.67,68 The interconnected conducting network of MWCNTs inside the insulating polymer matrix beneficially conducts charge through hoping, and generates conduction losses. Polarisation loss, which is the second component of total dielectric loss, mainly originates due to the interfacial polarisation of heterogeneous dielectric materials. As spherical-shaped Fe3O4 nanomaterials are highly dispersed compared with others, so they eventually possess maximum distribution of heterogeneous dielectric materials along with conducting MWCNTs, which leads to enhancement of the interfacial polarisation values. On the other hand, eddy current loss is the main cause of generating magnetic permeability loss in the gigahertz frequency region.67 For the estimation of the dielectric and magnetic lossy characteristics (ε′′ and μ′′) of the materials, dielectric and magnetic tangent (tan
δ) is usually calculated, where tan
δε= ε′′/ε′ and tan
δμ= μ′′/μ′.69 Therefore, with increasing tan
δ values, the relative permeability and permittivity parameter also increase. To elucidate the mechanism of shielding in the hybrid system, where both conducting and magnetic nanoparticles are incorporated, we calculated the consolidated loss tangent values (tan
δε + tan
δμ). A significant enhancement was observed in loss parameters after the addition of Fe3O4 nanoparticles in the blend, which clearly suggests a change in SET values (Fig. 10A). This is principally due to the addition of magnetic loss parameters with the dielectric loss parameter. Conversely, dielectric loss is also improved due to enhancement in interfacial polarisation and conduction loss.70 Despite the fact that high saturation magnetisation can drive stronger permeability loss in the blend, better dispersion of magnetic nanoparticles actually controls the eddy current loss throughout the matrix. Therefore, NS1 and NS2 show better consolidated loss parameters, accounting for enhanced interfacial polarisation and magnetic loss due to better dispersion in the matrix; whereas larger-sized Fe3O4 nanoparticles (NCu, NC and NF) exhibit lesser consolidated loss parameters due to poor dispersion in the matrix. In addition, the attenuation constant (α), which is defined as the ability of EM absorption, can also be evaluated by the corresponding permittivity and permeability parameters. The attenuation constant can express as follows (eqn (7)):66 |  | (7) |
where, c is the speed of light. As is evident, to achieve a higher attenuation ability in the materials, magnetic and dielectric losses should be as high as possible. Fig. 10B shows that the attenuation constant is increased significantly after addition of magnetic nanoparticles into the blend, as expected. Further enhancement of attenuation constant scales up with the dispersion of Fe3O4 nanoparticles. Therefore, the addition of two different characteristic nanoparticles to the blends, which offers a synergistic contribution of dielectric and magnetic components, simultaneously enhances the loss parameters and attenuation constant.23 The dispersion of nanoparticles inside the polymer matrix is also a prominent factor which enhances the synergistic effect to a significant extent. In addition, impedance matching of the shield material and air also helps to attenuate the incident EM wave by minimising the surface reflection. To achieve this, the characteristic impedance of the shield should be equal/close to that of free space.67 The characteristic impedance is determined by the ratio of the real part of complex permittivity to permeability. If the real part of the complex permittivity is much higher than the real part of the complex permeability, most of the incident electromagnetic waves will be reflected off the surface due to low surface resistance, rather than penetrating into the shield.67 From Fig. 10C, it is observed that inclusion of heterogeneous materials reduces this value in comparison with exclusively MWCNT-containing blend. The incorporation of Fe3O4 nanoparticles reduces the bulk electrical conductivity of the composites, as discussed earlier, and has a direct consequence on the real part of the permittivity. On the other hand, dispersion-induced magnetic permeability governs the total impedance matching phenomena. Therefore, when the incoming electromagnetic waves interact with the shield material, most of the radiation encounters the inner surface of the shield material, with very little surface reflection. The interacted EM waves come across a variety of microscopic boundaries due to various inclusions that constitute the heterostructure. Therefore, the subsequent variation of the local field at such a heterostructure has a considerable effect on the final absorption by the material as absorption depends quadratically on the electric field intensity.18 Maxwell–Wagner–Siller polarisation suggests charge accumulation at the interface of two different dielectric materials and the dissipation of whole energies to heat synergistically. Therefore, better dispersion results in a more heterostructure medium that accumulates more charge which eventually dissipates as heat.
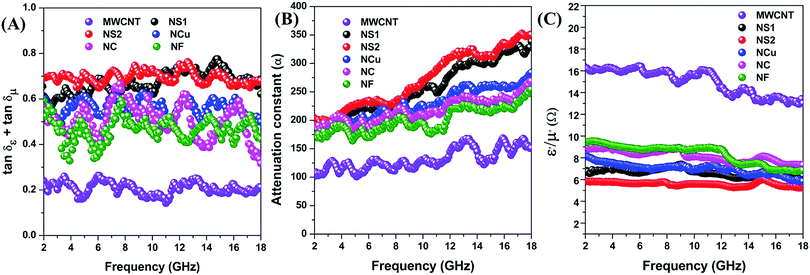 |
| Fig. 10 (A) Total tan loss parameters, (B) attenuation constant and (C) impedance matching of various blends. | |
The third shielding mechanism is multiple reflection; this represents the shielding originated by the reflection from the inner surface of the shield material. Typically, this type of reflection decreases the overall SE when the thickness of the shield material is thinner than the skin depth, and can be ignored when the shield is thicker than the skin depth. Generally, the strength of an EM wave decreases exponentially as it travels through the conductive material, and, at a certain distance, the electric field drops to 1/e, which is defined as the skin depth of that material. Theoretically, it can be calculated by this equation;
.2 Therefore, the interdependence in the blend conductivity and magnetic permeability is the governing factor for calculating the skin depth of the material. Evaluation of skin depth from scattering parameters shows an inverse proportionality with the absorption of the shield materials;
, where t is the thickness of the material. Fig. 11A clearly illustrates that the addition of magnetic nanoparticles along with conducting nanomaterials has a significant effect on altering the blends skin depth value and further indicates a boost in shielding by absorption. Here the dispersion of nanomaterials is also crucial as it controls the conductivity and permeability parameters effectively. The effect of the shield thickness has been evaluated at a frequency of 18 GHz for all samples. It is clear that below skin depth, the SE will reduce drastically due to the multiple reflections. Besides, the lack of interconnected conducting mesh, which is randomly placed in the system, also results in lowering of SE.71 As the skin depth is very high when the blend contains only MWCNTs, the shielding efficiency values gradually decrease with a faster rate when the thickness is decreased from 5 mm to 1 mm (Fig. 11B). Incorporation of magnetic nanoparticles mainly reduces the skin depth. As the dispersion of Fe3O4 nanoparticles scales up with the skin-depth value, the highly dispersed NS2 nanoparticles show even better SE at lower thickness (Table 2).
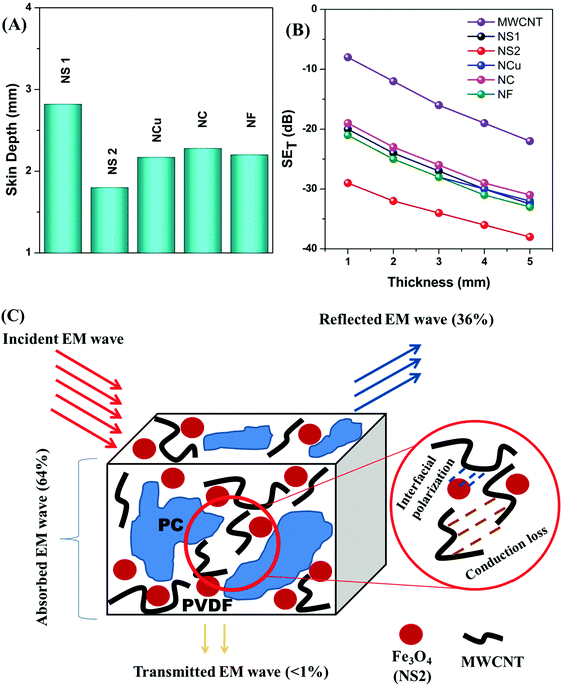 |
| Fig. 11 (A) Skin depth, (B) thickness-dependent shielding effectiveness of various blends and (C) mechanism of shielding. | |
Table 2 SE of various fillers containing PC/PVDF blend at 18 GHz
Sr. no. |
Fillers concentration (wt%) |
SET (dB) |
1 |
NS1, NS2, NCu, NC, NF (separately) 3 wt% |
0 |
2 |
MWCNT 3 wt% |
−21 |
3 |
MWCNT 3 wt% + NS1 3 wt% |
−32 |
4 |
MWCNT 3 wt% + NS2 3 wt% |
−38 |
5 |
MWCNT 3 wt% + NCu 3 wt% |
−30 |
6 |
MWCNT 3 wt% + NC 3 wt% |
−31 |
7 |
MWCNT 3 wt% + NF 3 wt% |
−33 |
However, the shielding mechanism is much more complex for the polymer blend with filler nanoparticles in comparison with homogeneous conductive barriers. The picture becomes further exacerbated when hybrid nanoparticles are present in the blend that provide large surface area of different dielectric materials for reflection, absorption and multiple reflection. Therefore, when the incoming electromagnetic waves interact with the polymeric shield material, most of the radiation encounters at the inner surface of the shield material, with very little surface reflection due to the lower conductivity values. The interacted EM waves come across a variety of microscopic boundaries associated with the inclusions that constitute the heterostructure. The heterostructures are generated due to the blending of magnetic nanomaterials along with the conducting fillers. Therefore, most of the incoming radiation interacts with the inner shield materials where heterogeneous dielectrics are present, subsequent variation of electric field intensities and charge accumulation at the interfacial position of heterogeneous dielectric materials enhance the absorption of EM waves and dissipate the energies into heat synergistically. In our case, the spherical nanoparticles of both NS1 and NS2 are shown to have a maximum dispersion ability in the matrix along with conducting MWCNTs. This, in turn, synergistically enhances both conductivity and different polarisation loss values, and finally improves the dissipation of incident EM energies. Interestingly, saturation magnetisation and magnetic permeability loss come into play when both NS1 and NS2 have equal dispersion ability. Thus, NS2 possesses the highest SE among others. On the other hand, NCu, NC and NF all show less SE due to poor dispersibility within the matrix. However, owing to their different sizes and shapes, saturation magnetisation varies, as discussed earlier, and shows the highest value in NF. This magnetisation eventually increases the magnetic permeability loss parameters, although the polarisation losses are minimal. Therefore, we observe a trend NF > NC > NCu in SE, which correlates with their saturation magnetisation and magnetic permeability values. The overall mechanism of the blend is schematically represented in Fig. 11C. Additionally, reduction in skin depth of the material also helps to sustain the SE at a lower thickness. This study sheds light, for first time, on the effect of size and shape of magnetic nanomaterials in the design of an efficient EM-shielding materials. Hindrance in the macromolecular pathway and the agglomeration are the two governing parameters for the efficient dispersion of nanomaterials. The relatively smaller size and moderate saturation magnetisation makes NS2 nanoparticles superior as a highly efficient EM absorber when blended with MWCNTs.
Conclusions
In summary, our study clearly demonstrates that by controlling the dispersion of nanofillers within the immiscible polymer blend PC/PVDF, an excellent EM absorber can be designed. Successful syntheses of various size- and shape-controlled magnetic Fe3O4 nanoparticles and their effect on EM-shielding applications were studied systematically. Moderate saturation magnetisation, high permittivity and permeability parameters ensured NS2 is a better magnetic filler component than others. However, saturation magnetisation and magnetic permeability take pivotal role when comparable dispersibility is observed. Additionally, presence of MWCNTs into the blend was instrumental in introducing efficient charge-transport properties. However, the change in the absorption-driven SE was demonstrated as a result of better impedance matching and interfacial polarisation of heterogeneous dielectric materials. The higher consolidated loss parameter and attenuation constant also corroborates the enhanced absorption at a lower skin depth. The maximum synergistic effect for both the nanofillers was achieved by dispersing the shape- and size-controlled Fe3O4 nanoparticles in the matrix.
Conflicts of interest
There are no conflicts to declare.
Acknowledgements
The authors gratefully acknowledge the financial support from DST-SERB (EMR/2016/001230), and INSA India. One of the authors, IA, acknowledges DST-SERB-National Post-Doctoral Fellowship (N-PDF) programme (Grant No. PDF/2016/000048) for financial assistance.
References
- D. Chung, Electromagnetic interference shielding effectiveness of carbon materials, Carbon, 2001, 39(2), 279–285 CrossRef CAS.
- M. H. Al-Saleh and U. Sundararaj, Electromagnetic interference shielding mechanisms of CNT/polymer composites, Carbon, 2009, 47(7), 1738–1746 CrossRef CAS.
- S. Geetha, K. Satheesh Kumar, C. R. Rao, M. Vijayan and D. Trivedi, EMI shielding: Methods and materials—A review, J. Appl. Polym. Sci., 2009, 112(4), 2073–2086 CrossRef CAS.
- S. P. Pawar, S. Biswas, G. P. Kar and S. Bose, High frequency millimetre wave absorbers derived from polymeric nanocomposites, Polymer, 2016, 84, 398–419 CrossRef CAS.
- Y. Yang, M. C. Gupta, K. L. Dudley and R. W. Lawrence, Novel carbon nanotube-polystyrene foam composites for electromagnetic interference shielding, Nano Lett., 2005, 5(11), 2131–2134 CrossRef CAS PubMed.
- F. Ren, H. Yu, L. Wang, M. Saleem, Z. Tian and P. Ren, Current progress on the modification of carbon nanotubes and their application in electromagnetic wave absorption, RSC Adv., 2014, 4(28), 14419–14431 RSC.
- S. R. Dhakate, K. M. Subhedar and B. P. Singh, Polymer nanocomposite foam filled with carbon nanomaterials as an efficient electromagnetic interference shielding material, RSC Adv., 2015, 5(54), 43036–43057 RSC.
- S. Biswas, G. P. Kar and S. Bose, Engineering nanostructured polymer blends with controlled nanoparticle location for excellent microwave absorption: a compartmentalized approach, Nanoscale, 2015, 7(26), 11334–11351 RSC.
- S. Biswas, G. P. Kar and S. Bose, Tailor-made distribution of nanoparticles in blend structure toward outstanding electromagnetic interference shielding, ACS Appl. Mater. Interfaces, 2015, 7(45), 25448–25463 CAS.
- H.-B. Zhao, Z.-B. Fu, H.-B. Chen, M.-L. Zhong and C.-Y. Wang, Excellent electromagnetic absorption capability of Ni/carbon based conductive and magnetic foams synthesized via a green one pot route, ACS Appl. Mater. Interfaces, 2016, 8(2), 1468–1477 CAS.
- X. Huang, J. Zhang, W. Rao, T. Sang, B. Song and C. Wong, Tunable electromagnetic properties and enhanced microwave absorption ability of flaky graphite/cobalt zinc ferrite composites, J. Alloys Compd., 2016, 662, 409–414 CrossRef CAS.
- S. Biswas, Y. Bhattacharjee, S. S. Panja and S. Bose, Graphene oxide co-doped with dielectric and magnetic phases as an electromagnetic wave suppressor, Mater. Chem. Front., 2017, 1(6), 1229–1244 RSC.
- I. Arief, S. Biswas and S. Bose, Tuning the Shape Anisotropy and Electromagnetic Screening Ability of Ultrahigh Magnetic Polymer and Surfactant-Capped FeCo Nanorods and Nanocubes in Soft Conducting Composites, ACS Appl. Mater. Interfaces, 2016, 8(39), 26285–26297 CAS.
- K. Singh, A. Ohlan, V. H. Pham, R. Balasubramaniyan, S. Varshney, J. Jang, S. H. Hur, W. M. Choi, M. Kumar and S. Dhawan, Nanostructured graphene/Fe3O4 incorporated polyaniline as a high performance shield against electromagnetic pollution, Nanoscale, 2013, 5(6), 2411–2420 RSC.
- G. P. Kar, S. Biswas, R. Rohini and S. Bose, Tailoring the dispersion of multiwall carbon nanotubes in co-continuous PVDF/ABS blends to design materials with enhanced electromagnetic interference shielding, J. Mater. Chem. A, 2015, 3(15), 7974–7985 CAS.
- Y. Qing, D. Min, Y. Zhou, F. Luo and W. Zhou, Graphene nanosheet-and flake carbonyl iron particle-filled epoxy–silicone composites as thin–thickness and wide-bandwidth microwave absorber, Carbon, 2015, 86, 98–107 CrossRef CAS.
- F. Qin and C. Brosseau, A review and analysis of microwave absorption in polymer composites filled with carbonaceous particles, J. Appl. Phys., 2012, 111(6), 4 CrossRef.
- M.-S. Cao, J. Yang, W.-L. Song, D.-Q. Zhang, B. Wen, H.-B. Jin, Z.-L. Hou and J. Yuan, Ferroferric oxide/multiwalled carbon nanotube vs polyaniline/ferroferric oxide/multiwalled carbon nanotube multiheterostructures for highly effective microwave absorption, ACS Appl. Mater. Interfaces, 2012, 4(12), 6949–6956 CAS.
- A. P. Singh, M. Mishra, D. P. Hashim, T. Narayanan, M. G. Hahm, P. Kumar, J. Dwivedi, G. Kedawat, A. Gupta and B. P. Singh, Probing the engineered sandwich network of vertically aligned carbon nanotube–reduced graphene oxide composites for high performance electromagnetic interference shielding applications, Carbon, 2015, 85, 79–88 CrossRef CAS.
- H. Hekmatara, M. Seifi, K. Forooraghi and S. Mirzaee, Synthesis and microwave absorption characterization of SiO2 coated Fe3O4–MWCNT composites, Phys. Chem. Chem. Phys., 2014, 16(43), 24069–24075 RSC.
- Y. Liu, D. Song, C. Wu and J. Leng, EMI shielding performance of nanocomposites with MWCNTs, nanosized Fe3O4 and Fe, Composites, Part B, 2014, 63, 34–40 CrossRef CAS.
- P. Bhattacharya, S. Sahoo and C. Das, Microwave absorption behaviour of MWCNT based nanocomposites in X-band region, eXPRESS Polym. Lett., 2013, 7, 212–223 CrossRef CAS.
- Z. Wang, L. Wu, J. Zhou, W. Cai, B. Shen and Z. Jiang, Magnetite nanocrystals on multiwalled carbon nanotubes as a synergistic microwave absorber, J. Phys. Chem. C, 2013, 117(10), 5446–5452 CAS.
- M.-M. Lu, M.-S. Cao, Y.-H. Chen, W.-Q. Cao, J. Liu, H.-L. Shi, D.-Q. Zhang, W.-Z. Wang and J. Yuan, Multiscale assembly of grape-like ferroferric oxide and carbon nanotubes: a smart absorber prototype varying temperature to tune intensities, ACS Appl. Mater. Interfaces, 2015, 7(34), 19408–19415 CAS.
- H. Hekmatara, M. Seifi and K. Forooraghi, Microwave absorption property of aligned MWCNT/Fe3O4, J. Magn. Magn. Mater., 2013, 346, 186–191 CrossRef CAS.
- Q. Liu, J. Dai, Z. Zi, A. Pang, Q. Liu, D. Wu and Y. Sun, Low temperature solution synthesis and microwave absorption properties of multiwalled carbon nanotubes/Fe3O4 composites, J. Low Temp. Phys., 2013, 170(5-6), 261–267 CrossRef CAS.
- Y. Xu, D. Zhang, J. Cai, L. Yuan and W. Zhang, Effects of multi-walled carbon nanotubes on the electromagnetic absorbing characteristics of composites filled with carbonyl iron particles, J. Mater. Sci. Technol., 2012, 28(1), 34–40 Search PubMed.
- Z. Wang, L. Wu, J. Zhou, Z. Jiang and B. Shen, Chemoselectivity-induced multiple interfaces in MWCNT/Fe3O4@ZnO heterotrimers for whole X-band microwave absorption, Nanoscale, 2014, 6(21), 12298–12302 RSC.
- C. Tsonos, N. Soin, G. Tomara, B. Yang, G. C. Psarras, A. Kanapitsas and E. Siores, Electromagnetic wave absorption properties of ternary poly(vinylidene fluoride)/magnetite nanocomposites with carbon nanotubes and graphene, RSC Adv., 2016, 6(3), 1919–1924 RSC.
- T. Wu, Y. Liu, X. Zeng, T. Cui, Y. Zhao, Y. Li and G. Tong, Facile hydrothermal synthesis of Fe3O4/C core–shell nanorings for efficient low-frequency microwave absorption, ACS Appl. Mater. Interfaces, 2016, 8(11), 7370–7380 CAS.
- J. Guo, H. Song, H. Liu, C. Luo, Y. Ren, T. Ding, M. A. Khan, D. P. Young, X. Liu and X. Zhang, Polypyrrole-interface-functionalized nano-magnetite epoxy nanocomposites as electromagnetic wave absorbers with enhanced flame retardancy, J. Mater. Chem. C, 2017, 5(22), 5334–5344 RSC.
- X. Huang, Y. Chen, J. Yu, J. Zhang, T. Sang, G. Tao and H. Zhu, Fabrication and electromagnetic loss properties of Fe3O4 nanofibers, J. Mater. Sci.: Mater. Electron., 2015, 26(6), 3474–3478 CrossRef CAS.
- N. Li, G.-W. Huang, Y.-Q. Li, H.-M. Xiao, Q.-P. Feng, N. Hu and S.-Y. Fu, Enhanced Microwave Absorption Performance of Coated Carbon Nanotubes by Optimizing the Fe3O4 Nanocoating Structure, ACS Appl. Mater. Interfaces, 2017, 9(3), 2973–2983 CAS.
- C.-l. Hou, T.-h. Li, T.-k. Zhao, H.-g. Liu, L.-h. Liu and W.-j. Zhang, Electromagnetic wave absorbing properties of multi-wall carbon nanotube/Fe3O4 hybrid materials, New Carbon Mater., 2013, 28(3), 184–190 CrossRef CAS.
- A. H. Lu, E. e. L. Salabas and F. Schüth, Magnetic nanoparticles: synthesis, protection, functionalization, and application, Angew. Chem., Int. Ed., 2007, 46(8), 1222–1244 CrossRef CAS PubMed.
- J. Rockenberger, E. C. Scher and A. P. Alivisatos, A new nonhydrolytic single-precursor approach to surfactant-capped nanocrystals of transition metal oxides, J. Am. Chem. Soc., 1999, 121(49), 11595–11596 CrossRef CAS.
- W. Cai and J. Wan, Facile synthesis of superparamagnetic magnetite nanoparticles in liquid polyols, J. Colloid Interface Sci., 2007, 305(2), 366–370 CrossRef CAS PubMed.
- G. Viau, F. Fievet-Vincent and F. Fievet, Nucleation and growth of bimetallic CoNi and FeNi monodisperse particles prepared in polyols, Solid State Ionics, 1996, 84(3-4), 259–270 CrossRef CAS.
- V. K. LaMer and R. H. Dinegar, Theory, production and mechanism of formation of monodispersed hydrosols, J. Am. Chem. Soc., 1950, 72(11), 4847–4854 CrossRef CAS.
- Y. Xia, Y. Xiong, B. Lim and S. E. Skrabalak, Shape-Controlled synthesis of metal nanocrystals: simple chemistry meets complex physics?, Angew. Chem., Int. Ed., 2009, 48(1), 60–103 CrossRef CAS PubMed.
- S. G. Kwon and T. Hyeon, Formation mechanisms of uniform nanocrystals via hot-injection and heat-up methods, Small, 2011, 7(19), 2685–2702 CrossRef CAS PubMed.
- M. C. Menard, K. J. Takeuchi, A. C. Marschilok and E. S. Takeuchi, Electrochemical discharge of nanocrystalline magnetite: structure analysis using X-ray diffraction and X-ray absorption spectroscopy, Phys. Chem. Chem. Phys., 2013, 15(42), 18539–18548 RSC.
- A. Patterson, The Scherrer formula for X-ray particle size determination, Phys. Rev., 1939, 56(10), 978 CrossRef CAS.
- M. Khairy, Synthesis, Characterization and Magnetic Properties of γ-irradiated and Unirradiated Magnetite Nanopowders, Int. J. Mater. Chem., 2013, 3(5), 106–111 Search PubMed.
- I. Arief and P. Mukhopadhyay, Amphiphilic triblock copolymer-assisted synthesis of hierarchical NiCo nanoflowers by homogeneous nucleation in liquid polyols, J. Magn. Magn. Mater., 2014, 372, 214–223 CrossRef CAS.
- D. Han, J. Wang and H. Luo, Crystallite size effect on saturation magnetization of fine ferrimagnetic particles, J. Magn. Magn. Mater., 1994, 136(1–2), 176–182 CrossRef CAS.
- S. Gee, Y. Hong, D. Erickson, M. Park and J. Sur, Synthesis and aging effect of spherical magnetite (Fe3O4) nanoparticles for biosensor applications, J. Appl. Phys., 2003, 93(10), 7560–7562 CrossRef CAS.
- A. Berkowitz, W. Schuele and P. Flanders, Influence of Crystallite Size on the Magnetic Properties of Acicular γ-Fe2O3 Particles, J. Appl. Phys., 1968, 39(2), 1261–1263 CrossRef CAS.
- J. Chen, C. Sorensen, K. Klabunde, G. Hadjipanayis, E. Devlin and A. Kostikas, Size-dependent magnetic properties of MnFe2O4 fine particles synthesized by coprecipitation, Phys. Rev. B: Condens. Matter Mater. Phys., 1996, 54(13), 9288 CrossRef CAS.
- J. M. D. Coey, Noncollinear spin arrangement in ultrafine ferrimagnetic crystallites, Phys. Rev. Lett., 1971, 27(17), 1140 CrossRef CAS.
- A. Berkowitz and J. Walter, Amorphous particles produced by spark erosion, Mater. Sci. Eng., 1982, 55(2), 275–287 CrossRef CAS.
- P. Zhao, L. Li, Y. Luo, Z. Lv, K. Xu, S. Li, J. Zhong, Z. Wang and Z. Peng, Effect of blend ratio on the morphology and electromagnetic properties of nanoparticles incorporated natural rubber blends, Composites, Part B, 2016, 99, 216–223 CrossRef CAS.
- S. Biswas, G. P. Kar and S. Bose, Simultaneous Improvement in Structural Properties and Microwave Shielding of Polymer Blends with Carbon Nanotubes, ChemNanoMat, 2016, 2(2), 140–148 CrossRef CAS.
- G. P. Kar, S. Biswas and S. Bose, Tailoring the interface of an immiscible polymer blend by a mutually miscible homopolymer grafted onto graphene oxide: outstanding mechanical properties, Phys. Chem. Chem. Phys., 2015, 17(3), 1811–1821 RSC.
- P. Pötschke, T. Fornes and D. Paul, Rheological behavior of multiwalled carbon nanotube/polycarbonate composites, Polymer, 2002, 43(11), 3247–3255 CrossRef.
- S. Bose, A. R. Bhattacharyya, A. R. Kulkarni and P. Pötschke, Electrical, rheological and morphological studies in co-continuous blends of polyamide 6 and acrylonitrile–butadiene–styrene with multiwall carbon nanotubes prepared by melt blending, Compos. Sci. Technol., 2009, 69(3), 365–372 CrossRef CAS.
- I. Aravind, K. H. Ahn, C. Ranganathaiah and S. Thomas, Rheology, morphology, mechanical properties and free volume of poly(trimethylene terephthalate)/polycarbonate blends, Ind. Eng. Chem. Res., 2009, 48(22), 9942–9951 CrossRef CAS.
- L. T. Vo and E. P. Giannelis, Compatibilizing poly(vinylidene fluoride)/nylon-6 blends with nanoclay, Macromolecules, 2007, 40(23), 8271–8276 CrossRef CAS.
- S. T. Knauert, J. F. Douglas and F. W. Starr, The effect of nanoparticle shape on polymer-nanocomposite rheology and tensile strength, J. Polym. Sci., Part B: Polym. Phys., 2007, 45(14), 1882–1897 CrossRef CAS.
- B. Shen, W. Zhai, M. Tao, J. Ling and W. Zheng, Lightweight, multifunctional polyetherimide/graphene@Fe3O4 composite foams for shielding of electromagnetic pollution, ACS Appl. Mater. Interfaces, 2013, 5(21), 11383–11391 CAS.
- R. Kumaran, S. D. Kumar, N. Balasubramanian, M. Alagar, V. Subramanian and K. Dinakaran, Enhanced Electromagnetic Interference Shielding in a Au–MWCNT Composite Nanostructure Dispersed PVDF Thin Films, J. Phys. Chem. C, 2016, 120(25), 13771–13778 CAS.
- S. Biswas, S. S. Panja and S. Bose, A novel fluorophore–spacer–receptor to conjugate MWNTs and ferrite nanoparticles to design an ultra-thin shield to screen electromagnetic radiation, Mater. Chem. Front., 2016, 1(1), 132–145 RSC.
- A. Ameli, Y. Kazemi, S. Wang, C. Park and P. Pötschke, Process-microstructure-electrical conductivity relationships in injection-molded polypropylene/carbon nanotube nanocomposite foams, Composites, Part A, 2017, 96, 28–36 CrossRef CAS.
- Y. Chen, H. B. Zhang, Y. Yang, M. Wang, A. Cao and Z. Z. Yu, High-Performance Epoxy Nanocomposites Reinforced with Three-Dimensional Carbon Nanotube Sponge for Electromagnetic Interference Shielding, Adv. Funct. Mater., 2016, 26(3), 447–455 CrossRef CAS.
- R. Lv, A. Cao, F. Kang, W. Wang, J. Wei, J. Gu, K. Wang and D. Wu, Single-crystalline permalloy nanowires in carbon nanotubes: Enhanced encapsulation and magnetization, J. Phys. Chem. C, 2007, 111(30), 11475–11479 CAS.
- D. Ding, Y. Wang, X. Li, R. Qiang, P. Xu, W. Chu, X. Han and Y. Du, Rational design of core-shell Co@ C microspheres for high-performance microwave absorption, Carbon, 2017, 111, 722–732 CrossRef CAS.
- S. Kumar, G. Datt, A. Santhosh Kumar and A. Abhyankar, Enhanced absorption of microwave radiations through flexible polyvinyl alcohol-carbon black/barium hexaferrite composite films, J. Appl. Phys., 2016, 120(16), 164901 CrossRef.
- B. Zhao, B. Fan, G. Shao, W. Zhao and R. Zhang, Facile synthesis of novel heterostructure based on SnO2 nanorods grown on submicron Ni walnut with tunable electromagnetic wave absorption capabilities, ACS Appl. Mater. Interfaces, 2015, 7(33), 18815–18823 CAS.
- D. Micheli, C. Apollo, R. Pastore and M. Marchetti, X-Band microwave characterization of carbon-based nanocomposite material, absorption capability comparison and RAS design simulation, Compos. Sci. Technol., 2010, 70(2), 400–409 CrossRef CAS.
- A. Chaudhary, S. Kumari, R. Kumar, S. Teotia, B. P. Singh, A. P. Singh, S. Dhawan and S. R. Dhakate, Lightweight and easily foldable MCMB-MWCNTs composite paper with exceptional electromagnetic interference shielding, ACS Appl. Mater. Interfaces, 2016, 8(16), 10600–10608 CAS.
- M. Rahaman, T. Chaki and D. Khastgir, Development of high performance EMI shielding material from EVA, NBR, and their blends: effect of carbon black structure, J. Mater. Sci., 2011, 46(11), 3989–3999 CrossRef CAS.
|
This journal is © the Partner Organisations 2017 |
Click here to see how this site uses Cookies. View our privacy policy here.