DOI:
10.1039/C6RA25793C
(Paper)
RSC Adv., 2017,
7, 2905-2912
Self-assembled PEG–carboxymethylcellulose nanoparticles/α-cyclodextrin hydrogels for injectable and thermosensitive drug delivery†
Received
25th October 2016
, Accepted 28th November 2016
First published on 12th January 2017
Abstract
Novel cellulose hydrogels based on the inclusion complex between α-cyclodextrin and binary-drug loaded nanoparticles (carboxymethylcellulose–betulinic acid/hydroxycamptothecine nanoparticles) were prepared in aqueous media for the first time. As the reversible supramolecular assembly behavior, the thermosensitive hydrogels showed distinctive reversible gel–sol transition properties related to structure at a particular temperature. The temperature of the gel–sol transition was decided by the concentration between the nanoparticles and α-cyclodextrin. In addition, the injectable drug delivery applications of the BA/HCPT-loaded hydrogel showed more effectively than that of free drugs and BA/HCPT-loaded nanoparticles for in vitro and in vivo studies. Such an injectable hydrogel with a unique structure and temperature responses would be a promising candidate for many intelligent delivery applications.
1. Introduction
Hydrogels are fascinating and versatile soft materials with three-dimensional cross-linked networks.1 Because of the attractive properties and strong application potential, hydrogels are widely employed in drug delivery, cell immobilization and histological engineering. As one of the branch in gel chemistry, supramolecular polymer hydrogels have aroused particularly intensive concern in consideration of stimuli-responsiveness to noncovalent units.2–4 Despite the huge potential of stimuli-responsive hydrogels, in many cases these systems have limited use as drug delivery platforms today, since they exhibit a relatively rapid release of hydrophilic drugs from the gel and inefficient hydrophobic drug loading.5
Polymeric nanoparticles have been a new kind of intelligent drug carrier for insoluble or amphiphilic drugs since 1984,6–8 which shows a lot of obvious advantages such as structural and functional diversity, high drug loading capacity, long plasma half-life, and tunable degradation time for controlled drug delivery.9,10 Indeed, polymeric nano-deliveries have many merit, but there are problems not we overlook such as the safety and stability.11 Recently, based on the properties of polymeric nanoparticles and supramolecular polymer hydrogels, nanocomposite hydrogels is designed and prepared by combing the nano-materials and hydrogels to improve the performance biomedicine fields.12,13 Supramolecular polypseudorotaxane (PPR), which is a classical model of supramolecular self-assembly, formed between cyclodextrins (CDs) and polymeric nanoparticles was thought to generate structural diversity as well as a major of property enhancements.14 Strong hydrogen bonds between the adjacent threaded CDs result in microcrystalline aggregation and then promote physical gel formation.15,16 It has been reported that the threading–dethreading process of PPR is a thermosensitive dynamic equilibrium, and the threading–dethreading transition temperature can be affected by the length of PEG chain and concentration of α-CD,17,18 which endows the PPR hydrogel with tunable temperature-responsive potentials. Furthermore, these nanocomposite hydrogels showed significant efficacy in inhibition of tumor growth in vivo.
We have previously developed carboxymethylcellulose–betulinic acid (CMC–BA) prodrugs.19 In this work, the amphiphilic prodrug CMC–BA and hydroxycamptothecine (HCPT) self-assembled into nanoparticles, and then with α-CD to form supramolecular hydrogels for injectable and thermosensitive drug delivery. Nanoparticles can promote the insoluble drug loading capacity of hydrogels, which can be modulated in a versatile way, with the modification of the nanoparticles. Moreover, the hybrid systems could enable routes of drug administration with limited systemic absorption but that can be useful in long term delivery systems. On the other hand, the injectable and thermosensitive hydrogels could be achieved by varying the concentrations of prodrugs and α-CD, and also provide nanoparticles with the clinically useful formulation needed for their clinical applications.
2. Experimental details
2.1. Reagents and materials
Sodium carboxymethylcellulose (CMC-Na) (degree of substitution = 0.82, MW = 275 kDa, FDA and EU food grade) was obtained from Sigma-Aldrich. Methoxy poly(ethylene glycol) carboxyl (M-PEG-COOH, MW = 2 kDa) was purchased from JenKem Technology Co., Ltd. (Beijing, China). Betulinic acid (BA) and hydroxycamptothecine (HCPT) were obtained from Preferred Biotechnology Co., Ltd (Sichuan, China). N-Hydroxysuccinimide (NHS), 4-dimethylaminopyridine (DMAP), and 1-ethyl-3-(3-dimethylaminopropyl)-carbodiimide HCl (EDC HCl) were purchased from Sigma Aldrich. Ultracentrifugation filters (Vivaspin MWCO 2 kDa, 3.5 kDa and 10 kDa) were bought from Fisher (Ottawa, ON, Canada).
Fetal bovine serum (FBS), Dulbecco's Modified Eagle's Medium (DMEM), penicillin, streptomycin, and Dulbecco's Phosphate-Buffered Saline (DPBS) were all purchased from Gibco. Cell-Counting Kit-8 (CCK-8) kit was received from the Dojindo Laboratories. The LLC cells were cultured by DMEM with 10% FBS, 1% streptomycin–penicillin and incubated in 5% CO2/95% air humidified atmosphere at 37 °C.
2.2. Animals and ethics
C57BL/6 mice (female) ranging in age from 6 to 7 weeks obtained from Beijing HFK Biosciece Co., Ltd. were maintained in the animal house of Institute of Process Engineering (Chinese Academy of Sciences, Beijing, China) in a controlled environment (22 ± 2 °C, 55 ± 5% relative humidity, a 12 h light/dark cycle). All of the animal experiments were in accordance with the guidelines set by the National Institutes of Health (NIH Publication No. 85-23, revised 1985) and were approved by the Experimental Animal Ethics Committee, Beijing.
2.3. Characterization
The chemical construction of prodrugs was dissolved in deuterated chloroform (CDCl3) and measured by 1H-NMR (DRX-600 Avance III spectrometer, Bruker). The rheological behaviours of the samples were analyzed by a rotational rheometer (Malvern Kinexus pro+). For the TEM (JEM-100CXa) analysis nanoparticles were diluted 50 to 100 times in deionized water, and then dropped onto the surface of a TEM copper grid with formvar film and naturally air dried before analysis at an acceleration voltage of 100 kV. The hydrogel samples were vacuum freeze dried and ground into a fine powder, and then placed on the conductive tape, coated with gold vapor for the SEM (Hitachi S-3400N II electron microscope) observations.
2.4. Formation of hydrogels
The CMC–BA prodrug was synthesized according to previously described methods.19 CMC–BA prodrug containing 29.46 ± 2.35 wt% BA was obtained. The hydrogels were prepared between α-CD and the PEG chains of CMC–BA via the host–guest inclusion complexation in two steps. The brief preparation process for the hydrogel is as follows: 0.2 g CMC–BA prodrug and 0.1 g HCPT thoroughly dissolve in 0.2 mL of DMSO and then cautiously added, dropwise, into a rapidly vortexing PBS solution (3.8 mL, pH 7.4). The CMC–BA/HCPT NPs solutions were obtained after continue beating for 1 min, then transferred to a MWCO 10 kDa cartridge, and dialyzed for 12 h with two exchanges of dialysate against PBS solution (pH 7.4, 100 mL), and freeze-dried to obtained the particles. Secondly, different concentration of α-CD (1 mL, 100 to 150 mg mL−1) was added into CMC–BA/HCPT NPs solutions (1 mL, 100 to 300 mg mL−1) under efficient stirring and deaerating. The hydrogel formed within different time.
2.5. Thermo-responsive behavior
The thermosensitive gel–sol transition of the hybrid solutions was determined by a vial inverted method. The aqueous solutions of each sample were placed in glass vial and then the vial was treated in a water-bath heater at specified temperature for 2 min. The thermosensitive gel–sol transition temperatures were detected by no flow (gel)–flow (sol) criterion. If no flow was observed for at least 1 min when a vial was inverted, the hydrogel was regarded as a gel state.
2.6. Temperature-mediated in vitro release kinetics studies
The CMC–BA/HCPT hydrogel was suspended in dialysis bag, and placed into PBS buffer (200 mL, pH 7.4), then incubated with shaking bath at 100 rpm at 25 °C or 37 °C, respectively. The BA and HCPT concentration was tested by obtaining periodic samples and determined by HPLC method using a reverse phase column (C18) (HCPT: 254 nm, 30
:
70 mixture (v/v) of acetonitrile–water with flow rate at 1.0 mL min−1; BA: 210 nm, 85
:
15 mixture (v/v) of acetonitrile–water with flow rate at 1.0 mL min−1). The amount of release was then calculated. Each sample was measured by three independent runs and calculated mean value. Drug loading content (DLC) can be calculated using the following equation: DLC (%) = (weight of loaded BA or HCPT/weight of hydrogel) × 100%.
2.7. Hemolysis assay
The hemolytic activity of polymer solutions was described as follows.20 Ten milliliters of fresh blood samples were collected from mice, and then added with EDTA-Na2 immediately to prevent coagulation. The red blood cells (RBCs) were collected by centrifugation at 1500 rpm for 10 min at 4 °C. After washing in ice-cold DPBS until the supernatant was clear, erythrocytes were diluted to 5 × 108 cells per mL in ice-cold DPBS. 1 mL (1 mg mL−1 and 0.1 mg mL−1) polymer sol sample or PEI25 K solution was mixed with 1 mL erythrocyte suspension. 1% Triton X-100 in DPBS and DPBS were used as positive control (100% lysis) and negative control (0% lysis), respectively. Samples were incubated under constant shaking for 1 h at 37 °C. After centrifugation at 1500 rpm for 10 min at 4 °C, supernatant was analyzed for hemoglobin release at 541 nm using an infinite M200 microplate spectrophotometer (Tecan, Switzerland). Hemoglobin release was calculated as (absorbance of polymer samples − absorbance of negative control)/(absorbance of positive control − absorbance of negative control) × 100%. Hemolysis was determined from three independent experiments.
2.8. In vitro cytotoxicity
The cell viabilities of different samples were evaluated by CCK-8 assay.21,22 Briefly, LLC cells were seeded in 180 μL DMEM (3 × 103 cells per well). Incubated after 24 h, the DMEM was completely removed and 200 μL of drug solutions were added to each well. Then, the cells were incubated with different drugs or materials constant temperature oven for 72 h. 20 μL of CCK-8 solution was added and continued to incubate for 1 h. Cell survival rate can be determine by calculating the absence values of the samples at 450 nm. 50% inhibitive concentration (IC50) was computed using the Boltzmann sigmoidal function from Origin® 8.6. Data were repeated for independent tests.
2.9. In vivo antitumor activity
3 × 106 LLC cells in 0.2 mL PBS were injected into the right axillary flank region of the mice to develop tumor xenografts. After tumor volume (TV) reaching 100 to 150 mm3, treatments were initiated and this day was determined to be the day 0. From this day, thirty-six laboratory mice bearing LLC tumors were divided into 6 groups randomly, which respectively includes (a) normal saline, (b) 10 mg kg−1 of free BA, (c) 10 mg kg−1 of free HCPT, (d) CMC–BA/HCPT NPs (10 mg HCPT-equivalent per kg), (d) CMC–BA/HCPT hydrogel (10 mg HCPT-equivalent per kg), and (e) α-CD (100 mg kg−1). The TV was measured every other day for 6 weeks or the implanted TV reached to 5000 mm3. Using vernier calipers, measured and calculated TV, relative tumor volume (RTV), and the percentage of tumor growth inhibition (% TGI) by the formula of TV = [length × (width)2]/2, RTV = TVt/TV0, TGI = [(C − T)/C] × 100%, where TVt and TV0 is the TV at specified time and prior to initial treatment, respectively. C and T is the mean TV of the control and treatment group, respectively, at a given time point.23,24
2.10. Statistical analysis
All experiments in this study were performed at least three times, and the data were expressed as the means standard deviation (SD). Statistical analyses were performed by analysis of variance (ANOVA). In all analyses, p < 0.05 was taken to indicate statistical significance.
3. Results and discussion
3.1. Characterization of the CMC–BA/HCPT NPs
As previously reported,19 the synthesis of CMC–BA prodrug is a reproducible procedure, with the optimal composition containing 29.46 ± 2.35 wt% BA (Fig. 1). The synthetic routes and chemical composition (1H-NMR) of CMC–BA prodrug were shown in Fig. S1 and S2.† CMC–BA/HCPT NPs prepared from this CMC–BA prodrug were 168.5 ± 8.1 nm containing 22.95 ± 2.77 wt% BA and 22.10 ± 2.16 wt% HCPT. Fig. 2a showed the TEM images of nanoparticles.
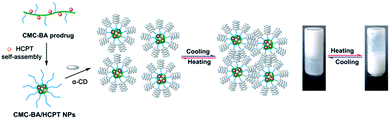 |
| Fig. 1 Schematic of the thermosensitive hydrogel made of CMC–BA/HCPT NPs and α-CD. | |
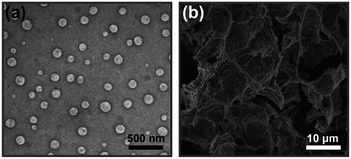 |
| Fig. 2 TEM images of CMC–BA/HCPT NPs (a) and SEM images of the CMC–BA/HCPT hydrogel being freeze-dried (b). | |
3.2. Effect of system concentration on the gel–sol transition temperature
The temperature-responsive hydrogel was fabricated by mixing CMC–BA/HCPT NPs solutions and α-CD molecules directly, as shown in Fig. 1. The PEG were hydrophilic and mainly offered random coil structure in CMC–BA/HCPT NPs solutions. After adding α-CD molecules, the hydrogels formed by the host–guest inclusion complexation between α-CD and the PEG chains outside the nanoparticles.25 Fig. 2b shows the SEM images of the dried CMC–BA/HCPT hydrogel. A typical porous structure was exhibited obviously from the obtained hydrogel, which is indispensable in drug delivery application allowing for drugs diffusion.26,27
We investigated the influence of the system concentration (α-CD and nanoparticles) on their thermo-responsive sol–gel transition behaviour. Fig. 3a and b showed the phase diagrams of CMC–BA/HCPT hydrogel formed between α-CD and CMC–BA/HCPT NPs with various concentrations. The gel–sol transition temperature (Tgel–sol) for different gels increased with the increase of the nanoparticles and α-CD concentration. Many studies have demonstrated that the process of forming PPR was summarized in the following five steps: (i) PEG chains and α-CD dispersion, (ii) PEG ends initial threading into α-CD cavities, (iii) α-CD sliding over the PEG chain, (iv) α-CD dethreading from the PEG chain, and (v) the final PPR precipitation.28 The process of forming PPR is a dynamic equilibrium, from which we can see that the significance of the concentration of α-CD. Certain concentration of α-CD in system could slow the step (iv) to form the PPR. As the concentration of α-CD was increased, the achievement of the step (iv) was harder therefore prolonged the gelation time. On the basis of the above results, the Tgel–sol of GEL-7 showed closer to the body temperature (Table 1). Therefore, the GEL-7 with a 1.98 wt% BA and 1.90 wt% HCPT loading efficiency was chosen for the following properties characterization and anti-tumor activity assay.
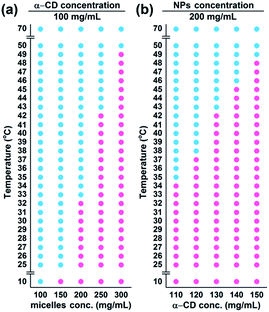 |
| Fig. 3 Thermosensitive sol–gel transition behavior. : sol, : gel. | |
Table 1 The gel–sol transition temperature and drug loading efficiency of the hydrogel
Gels |
Nanoparticles (mg mL−1) |
α-CD (mg mL−1) |
Tgel–sol (°C) |
DLEBA (wt%) |
DLEHCPT (wt%) |
GEL-1 |
100 |
100 |
— |
1.04 |
1.00 |
GEL-2 |
150 |
100 |
25 |
1.53 |
1.47 |
GEL-3 |
200 |
100 |
33 |
2.00 |
1.92 |
GEL-4 |
250 |
100 |
43 |
2.44 |
2.35 |
GEL-5 |
300 |
100 |
50 |
2.87 |
2.76 |
GEL-6 |
200 |
110 |
35 |
1.99 |
1.91 |
GEL-7 |
200 |
120 |
38 |
1.98 |
1.90 |
GEL-8 |
200 |
130 |
43 |
1.97 |
1.90 |
GEL-9 |
200 |
140 |
46 |
1.96 |
1.89 |
GEL-10 |
200 |
150 |
49 |
1.95 |
1.88 |
3.3. Viscoelastic behaviour of CMC–BA/HCPT hydrogel
Fig. 4a and b showed the viscoelastic behaviour of the hydrogel which was measured by dynamic mechanical measurements. As a kind of elastomeric hydrogel, the storage modulus (G′) and the loss modulus (G′′) of gel-2 increased and decreased slowly, respectively, in the range of whole frequency. And as expected, the G′ is about one order of magnitude higher than the G′′ (Fig. 4a). Moreover, when the shear rate increased, the viscosity of the hydrogel dramatically dropped (Fig. 4b). The viscosity showed a remarkable shear-thinning effect. The viscosity showed a remarkable shear-thinning effect. The uniformly distributed hydrophobic nanoparticles core and the hydrophobic–hydrophobic interactions between the HCPT and BA molecules inner shell of the nanoparticles can improve the strength of the hydrogels to a certain extent.29 Thus, for these viscoelasticity, the CMC–BA/HCPT hydrogel can be an efficient injectable material for drug delivery.
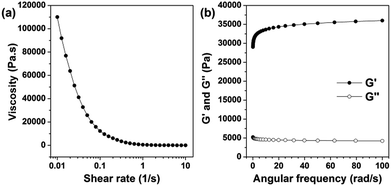 |
| Fig. 4 (a) Dynamic and (b) steady rheological behaviors of the GEL-7. | |
3.4. In vitro release of BA and HCPT
In vitro release kinetic of BA and HCPT from CMC–BA/HCPT hydrogel which affected by temperature was quantified by HPLC analysis (Fig. 5a and b). When the incubation temperature increases from 25 °C to 37 °C, hydrogel release rates showed obvious accelerating phenomenon. This accelerated release was attributed to the sol state of the temperature responsive hydrogel. These release properties can be a benefit to the enhanced cytotoxicity in vivo and stable in vitro. However, the hydrogels rapidly hydrolyzed and released the drugs in the presence of esterase (abundant in cytoplasm).30 Therefore, CMC–BA/HCPT hydrogel in sol state were prodrugs for intracellular release of BA.
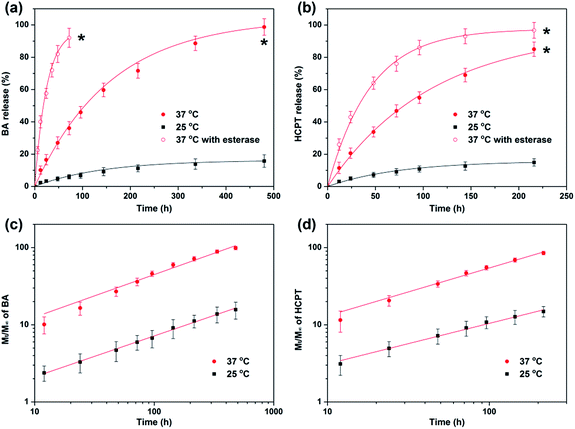 |
| Fig. 5 BA (a) and HCPT (b) release from GEL-7. Plots of log(Mt/M∞) against log t for BA (c) and HCPT (d) release from the GEL-7 at 25 °C and 37 °C. | |
An empirical power equation has been proposed to explain the behaviors of drug release.31,32 The equation is written as: log(Mt/M∞) = n
log
t + log
k, where Mt and M∞ are the cumulative amount of drug released at time t and infinite time, respectively, k is a constant incorporating structural and geometric characteristics of the device, and n is the release exponent, indicative of the mechanism of drug release.
When n has value 0.45 or 0.89, the drug release behavior was called Fickian diffusion or case-II transport, and indicated diffusion-controlled release or swelling-controlled release, respectively.33 When n is between 0.45 and 0.89, the drug release behavior was called anomalous transport which can be viewed as the superposition of both phenomena.
log(Mt/M∞) versus log
t of the experimental data has been plotted according to the equation. Fig. 5c and d shows a typical plot of log(Mt/M∞) against log
t in hydrogel at 25 °C and 37 °C. Good linear relation indicated that the Peppas's equation is suitable to this hydrogel system. By these plots, Table 2 shows the values of n and log
k for the CMC–BA/HCPT hydrogel. The n values for BA and HCPT are all much smaller than 0.89 and greater than 0.45, indicating that the release is anomalous transport. The release mechanism suggests that the nanoparticles have a influence on the drugs in their release process.32 The values of log
k for BA and HCPT as significantly increased as the temperature increased which indicated thermosensitivity of the release rate.
Table 2 Caption release exponent (n), rate constant (log
k), and correlation coefficient (R2) for hydrogel9; T = 25 °C and 37 °C
Drug |
T (°C) |
log k |
n |
R2 |
BA |
25 |
−0.20 |
0.53 |
0.99 |
37 |
0.55 |
0.55 |
0.99 |
HCPT |
25 |
−0.03 |
0.52 |
0.99 |
37 |
0.62 |
0.50 |
0.99 |
3.5. Hemolysis study
Harmful interaction of nanoparticles and hydrogel with RBCs must be avoided when these drug formulations enter the bloodstream.34 Hemolysis was detected and the results were shown in Fig. 6. As the positive control, Triton X-100 induced full hemoglobin release. Drug-loading nanoparticles and hydrogel (gel-2) at different concentrations showed a extremely low hemoglobin release (<5%), which comparable to blank values and significantly lower than the same concentrations of PEI25 K. Despite HCPT and BA has certain toxicity to the RBCs in earlier studies,35,36 the hydrogel has been released small amounts of free drugs during the short incubation period, suggesting the reliable security of the hydrogel.
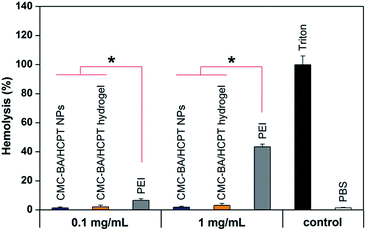 |
| Fig. 6 Hemolysis assay of nanoparticles and hydrogel (GEL-7). | |
3.6. In vitro cytotoxicity
Cytotoxicity of the GEL-7 on LLC cells was determined by the CCK-8 assay. Previous studies have shown that cytotoxicity is related with duration of drug treatment. In order to compare the effect of drug formulations, the IC50 after 72 h were obtained from survival curves as shown in Fig. 7. The results showed that the IC50 of the sample is in the order free BA > free HCPT > CMC–BA/HCPT NPs > CMC–BA/HCPT hydrogel.
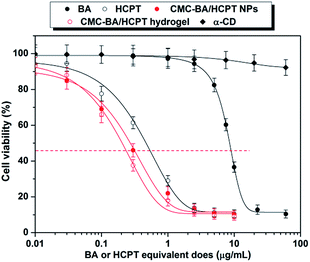 |
| Fig. 7 Cell viability and IC50 of LLC cells treated with different concentrations of the samples. | |
Compared to the free BA treatment, the CMC–BA/HCPT NPs and hydrogel (GEL-7) treatment was 29.7 and 40.5 times more toxic than LLC, respectively. Compared to the free HCPT, the CMC–BA/HCPT NPs and hydrogel (GEL-7) treatment was 1.7 and 2.4 times more toxic than LLC, respectively (Table 3). The increased toxicities of CMC–BA/HCPT NPs and GEL-7 were approximately related to the slow release of the drugs. Beyond the advantages of slow release, the combined treatment with BA and HCPT can improve in vitro therapeutic effectiveness significantly versus a single drug. Both HCPT and BA are potent Topoisomerase (Top) inhibitors, but HCPT just interferes with the action of Top I. Certain clinical limitations such as resistance of cancer cells impair its clinical application.15 It has been proven that the increase of Top II activity occurs in cancer cells resistant to HCPT.16 BA has been reported to be a catalytic inhibitor of TOP I and II activity. Top II inhibitor shows a collateral cytotoxicity on the HCPT adapted cancer cells. Therefore, the concomitant use of both Top I and Top II inhibitors might elicit synergistic effects and prevent the emergence of drug resistance. We evaluate the synergistic effects between BA and HCPT in GEL-7 by applying the combination index (CI) used in the combination treatment. CI = BAcombined/BAsingle + HCPTcombined/HCPTsingle, whereby BAcombined and HCPTcombined represent the IC50 of drugs, and BAsingle and HCPTsingle represents single drug IC50. The CI value lesser or larger than 1 indicated a synergistic or an antagonistic effect, respectively. GEL-7 IC50 of LLC cells was 0.22 μg mL−1, the calculated CI value of BA and HCPT in GEL-7 were 0.45, which suggested that GEL-7 achieve the cooperative action of BA and HCPT.37
Table 3 In vitro cytotoxicity analysis
Compound |
IC50, μg mL−1 |
α-CD |
— |
BA |
8.92 (0.50287) |
HCPT |
0.52 (0.02936) |
CMC–BA/HCPT NPs |
0.30 (0.02015) |
CMC–BA/HCPT hydrogel (GEL-7) |
0.22 (0.01357) |
3.7. In vivo antitumor activity
Although various kinds of drug delivery systems emerged endlessly, localized therapy is a very effective cure for cancers.38–40 Accordingly, we investigated GEL-7 for localized BA and HCPT delivery by an effective and simple peritumoral injection strategy. Tumor-bearing mice treated with GEL-7 achieved higher survival rate than the other groups (Fig. 8b). On the other hand, the treatment of saline (negative control) or α-CD showed hardly therapeutic effect. Injection of free BA and HCPT could have a certain degree of efficacy, but only days later the tumor was growing uncontrollably. Oppositely, the injection of the nanoparticles and GEL-7, particularly GEL-7, inhibited the growth of tumor obviously during this experimental period (Table 4, Fig. 8a). And best of all, no signs of systemic toxicity were found in the monitored general behavior, appetite and body weight (Fig. 8c). The better therapeutic effects of the GEL-7 may be anticipated on the basis of the following reasons: (1) GEL-7 have excellent sustained release of drugs, (2) nanoparticles in GEL-7 has the appropriate size (∼150 nm), which can lead more nanoparticles to be accumulated at the tumor site via the EPR effect,41 and (3) drug-loaded hydrogel and nanoparticles can improve the drugs poor solubility to some extent.42 All these results suggest that the BA and HCPT-loaded gel-2 could be a safe and effect candidate formulation for delivery anticancer drugs and suppression of tumor growth.
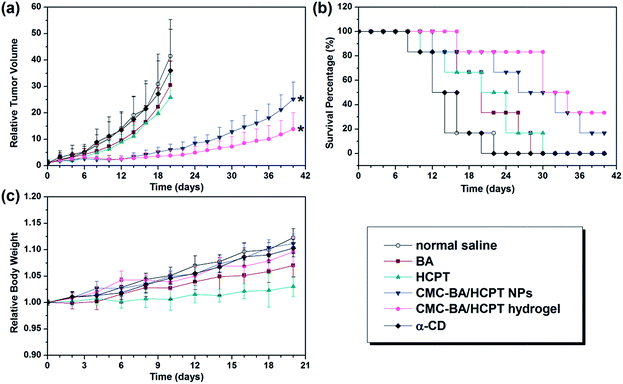 |
| Fig. 8 In vivo antitumor activity of the samples in the subcutaneous mouse model of LLC. (a) Tumor volumes of mice during treatment with different groups. (b) Survival of mice in different treatment. (c) The animal weights were recorded once per week and expressed over the 20 day observation. | |
Table 4 The antitumor efficacy of different groups
Compound |
Mean TV ± SDa (mm3) |
RTVa |
TGIa (%) |
Curesb (%) |
20 days of mean TV, RTV, and TGI data. 28 days of cures data. |
Normal saline |
5436 ± 1808 |
41.5 ± 13.8 |
0 |
0 |
α-CD |
4968 ± 2153 |
36.0 ± 15.6 |
8.6 |
0 |
BA |
3690 ± 1101 |
30.5 ± 9.1 |
32.1 |
16.7 |
HCPT |
3328 ± 1109 |
25.8 ± 8.6 |
38.8 |
16.7 |
CMC–BA/HCPT NPs |
781 ± 282 |
6.1 ± 2.2 |
85.6 |
50.0 |
GEL-7 |
502 ± 238 |
3.8 ± 1.8 |
90.8 |
83.3 |
4. Conclusion
An injectable and thermosensitive drug delivery system from CMC–BA/HCPT NPs/α-CD hydrogel has been prepared by a combination of the host–guest inclusion complexation between α-CD and the PEG chains outside the nanoparticles. The structure and thermosensitivity of PPR could be controlled simply by varying the concentration of the nanoparticles and α-CD. GEL-7, the Tgel–sol closer to the body temperature, was chosen for the following studies. The obtained release profiles of hydrogel are well fitted by the classical empirical power law. In addition, this injectable hydrogel showed appealing properties as an anticancer drug delivery system consist of simple preparation, good thermosensitive, high loading capacity, favorable stability, long release profile, good antitumor activity in vitro and in vivo, and low systemic toxicity. This work may promote the nano-materials and injectable hydrogel for drug delivery.
Acknowledgements
This work was financially supported by the State Key Laboratory of Tree Genetics and Breeding, State Key Laboratory of Pulp and Paper Engineering (201611), Jiangsu Province Biomass Energy and Materials Laboratory in Institute of Chemical Industry of Forest Products, CAF (JSBEM201601).
Notes and references
- H. Kuang, H. He, Z. Zhang, Y. Qi, Z. Xie, X. Jing and Y. Huang, J. Mater. Chem. B, 2014, 2, 659–667 RSC.
- E. A. Appel, J. del Barrio, X. J. Loh and O. A. Scherman, Chem. Soc. Rev., 2012, 41, 6195–6214 RSC.
- K. Miyamae, M. Nakahata, Y. Takashima and A. Harada, Angew. Chem., Int. Ed., 2015, 54, 8984–8987 CrossRef CAS PubMed.
- Y. Guan, J. Bian, F. Peng, X.-M. Zhang and R.-C. Sun, Carbohydr. Polym., 2014, 101, 272–280 CrossRef CAS PubMed.
- S. Merino, C. Martin, K. Kostarelos, M. Prato and E. Vazquez, ACS Nano, 2015, 9, 4686–4697 CrossRef CAS PubMed.
- Y. Miura, T. Takenaka, K. Toh, S. Wu, H. Nishihara, M. R. Kano, Y. Ino, T. Nomoto, Y. Matsumoto, H. Koyama, H. Cabral, N. Nishiyama and K. Kataoka, ACS Nano, 2013, 7, 8583–8592 CrossRef CAS PubMed.
- H. Cabral and K. Kataoka, J. Controlled Release, 2014, 190, 465–476 CrossRef CAS PubMed.
- M. Talelli, M. Barz, C. J. F. Rijcken, F. Kiessling, W. E. Hennink and T. Lammers, Nano Today, 2015, 10, 93–117 CrossRef CAS PubMed.
- N. Kamaly, Z. Xiao, P. M. Valencia, A. F. Radovic-Moreno and O. C. Farokhzad, Chem. Soc. Rev., 2012, 41, 2971–3010 RSC.
- H. Wei, R.-X. Zhuo and X.-Z. Zhang, Prog. Polym. Sci., 2013, 38, 503–535 CrossRef CAS.
- R. Duncan, Nat. Rev. Drug Discovery, 2003, 2, 347–360 CrossRef CAS PubMed.
- A. K. Gaharwar, N. A. Peppas and A. Khademhosseini, Biotechnol. Bioeng., 2014, 111, 441–453 CrossRef CAS PubMed.
- N. Annabi, A. Tamayol, J. A. Uquillas, M. Akbari, L. E. Bertassoni, C. Cha, G. Camci-Unal, M. R. Dokmeci, N. A. Peppas and A. Khademhosseini, Adv. Mater., 2014, 26, 85–124 CrossRef CAS PubMed.
- P. Thoniyot, M. J. Tan, A. A. Karim, D. J. Young and X. J. Loh, Adv. Sci., 2015, 2, 1400010 CrossRef PubMed.
- J. Li and X. J. Loh, Adv. Drug Delivery Rev., 2008, 60, 1000–1017 CrossRef CAS PubMed.
- A. Harada, A. Hashidzume, H. Yamaguchi and Y. Takashima, Chem. Rev., 2009, 109, 5974–6023 CrossRef CAS PubMed.
- J. Li, X. Ni and K. W. Leong, J. Biomed. Mater. Res., Part A, 2003, 65, 196–202 CrossRef PubMed.
- M. Ceccato, P. Lo Nostro and P. Baglioni, Langmuir, 1997, 13, 2436–2439 CrossRef CAS.
- L. Dai, K.-F. Liu, C.-L. Si, J. He, J.-D. Lei and L.-Q. Guo, J. Mater. Chem. B, 2015, 3, 6605–6617 RSC.
- R. Reul, J. Nguyen and T. Kissel, Biomaterials, 2009, 30, 5815–5824 CrossRef CAS PubMed.
- D. Ni, H. Ding, S. Liu, H. Yue, Y. Bao, Z. Wang, Z. Su, W. Wei and G. Ma, Small, 2015, 11, 2518–2526 CrossRef CAS PubMed.
- L. Dai, K. Liu, C. Si, L. Wang, J. Liu, J. He and J. Lei, J. Mater. Chem. B, 2016, 4, 529–538 RSC.
- K. Dong, Z. Liu, Z. Li, J. Ren and X. Qu, Adv. Mater., 2013, 25, 4452–4458 CrossRef CAS PubMed.
- A. J. Souers, J. D. Leverson, E. R. Boghaert, S. L. Ackler, N. D. Catron, J. Chen, B. D. Dayton, H. Ding, S. H. Enschede, W. J. Fairbrother, D. C. S. Huang, S. G. Hymowitz, S. Jin, S. L. Khaw, P. J. Kovar, L. T. Lam, J. Lee, H. L. Maecker, K. C. Marsh, K. D. Mason, M. J. Mitten, P. M. Nimmer, A. Oleksijew, C. H. Park, C.-M. Park, D. C. Phillips, A. W. Roberts, D. Sampath, J. F. Seymour, M. L. Smith, G. M. Sullivan, S. K. Tahir, C. Tse, M. D. Wendt, Y. Xiao, J. C. Xue, H. Zhang, R. A. Humerickhouse, S. H. Rosenberg and S. W. Elmore, Nat. Med., 2013, 19, 202–208 CrossRef CAS PubMed.
- X. Ma and Y. Zhao, Chem. Rev., 2015, 115, 7794–7839 CrossRef CAS PubMed.
- J. He, M. Zhang and P. Ni, Soft Matter, 2012, 8, 6033–6038 RSC.
- X. Huang and C. S. Brazel, J. Controlled Release, 2001, 73, 121–136 CrossRef CAS PubMed.
- M. Arunachalam and H. W. Gibson, Prog. Polym. Sci., 2014, 39, 1043–1073 CrossRef CAS.
- H. Zou, W. Guo and W. Yuan, J. Mater. Chem. B, 2013, 1, 6235–6244 RSC.
- Y. Shen, E. Jin, B. Zhang, C. J. Murphy, M. Sui, J. Zhao, J. Wang, J. Tang, M. Fan, E. Van Kirk and W. J. Murdoch, J. Am. Chem. Soc., 2010, 132, 4259–4265 CrossRef CAS PubMed.
- P. L. Ritger and N. A. Peppas, J. Controlled Release, 1987, 5, 23–36 CrossRef CAS.
- J. Siepmann and N. A. Peppas, Adv. Drug Delivery Rev., 2001, 48, 139–157 CrossRef CAS PubMed.
- P. Colombo, R. Bettini, P. L. Catellani, P. Santi and N. A. Peppas, Eur. J. Pharm. Sci., 1999, 9, 33–40 CrossRef CAS PubMed.
- J. G. Schellinger, J. A. Pahang, R. N. Johnson, D. S. H. Chu, D. L. Sellers, D. O. Maris, A. J. Convertine, P. S. Stayton, P. J. Horner and S. H. Pun, Biomaterials, 2013, 34, 2318–2326 CrossRef CAS PubMed.
- M. Gao, P. M. Lau and S. K. Kong, Arch. Toxicol., 2014, 88, 755–768 CAS.
- Z. Su, J. Niu, Y. Xiao, Q. Ping, M. Sun, A. Huang, W. You, X. Sang and D. Yuan, Mol. Pharmaceutics, 2011, 8, 1641–1651 CrossRef CAS PubMed.
- T.-C. Chou, Cancer Res., 2010, 70, 440–446 CrossRef CAS PubMed.
- N. Bhattarai, J. Gunn and M. Zhang, Adv. Drug Delivery Rev., 2010, 62, 83–99 CrossRef CAS PubMed.
- O. P. Varghese, W. Sun, J. Hilborn and D. A. Ossipov, J. Am. Chem. Soc., 2009, 131, 8781–8783 CrossRef CAS PubMed.
- Y. Yang, J. Wang, X. Zhang, W. Lu and Q. Zhang, J. Controlled Release, 2009, 135, 175–182 CrossRef CAS PubMed.
- H. Maeda, Adv. Drug Delivery Rev., 2015, 91, 3–6 CrossRef CAS PubMed.
- O. P. Medina, N. Pillarsetty, A. Glekas, B. Punzalan, V. Longo, M. Gönen, P. Zanzonico, P. Smith-Jones and S. M. Larson, J. Controlled Release, 2011, 149, 292–298 CrossRef CAS PubMed.
Footnote |
† Electronic supplementary information (ESI) available. See DOI: 10.1039/c6ra25793c |
|
This journal is © The Royal Society of Chemistry 2017 |
Click here to see how this site uses Cookies. View our privacy policy here.