DOI:
10.1039/C6RA25906E
(Paper)
RSC Adv., 2017,
7, 914-918
Phototriggered base proliferation: a powerful 365 nm LED photoclick tool for nucleophile-initiated thiol-Michael addition reaction
Received
27th October 2016
, Accepted 14th November 2016
First published on 24th November 2016
Abstract
Photocaged amines (PCAs) can allow a spatiotemporal control of the highly versatile and widely implemented nucleophile-catalyzed thiol-Michael addition reaction. However, a major challenge with most PCAs is that their relatively low quantum yields easily lead to low photosensitivity, especially under the radiation of light-emitting diodes (LEDs). In this paper, the phototriggered base proliferation (PBP) reaction as a powerful 365 nm LED photoclick tool is presented for the nucleophile-initiated thiol-Michael addition reaction. Compared to the PCA system, the advantages of this approach lie in its enhanced photosensitivity, increased reaction rate and elevated conversion. Finally, due to the persistent interactions of the produced longeval amine, remarkable post conversion was thus initially achieved after irradiation.
Introduction
Over the past decade, click chemistry has continuously received a significant amount of attention in the design and synthesis of complex, highly functional molecules in areas such as polymer and material development, small molecule organic chemistry and drug discovery.1,2 One of the most attractive organic reactions, thiol–ene addition, has become popular owing to its ease of implementation, non-toxicity and high efficiency.3 Typically, thiol–ene addition reactions can proceed by radical-mediated anti-Markovnikov addition. The thiyl radical across the C
C bond yields an intermediate carbon-centered radical, which can not only undergo the desired chain transfer reaction but also add another radical across the C
C bond; i.e., the radical can propagate, resulting in oligomers/polymer as contaminants in the final product. It is obvious that one drawback of the radical-mediated thiol–ene addition reactions is the nonquantitative formation of target thioether with α,β-unsaturated electron-deficient alkenes, such as acrylates and acrylamides.4
Much research has been directed toward the use of alternative amines or nucleophile-catalyzed Michael addition with quantitative conversions.4–10 To further achieve spatiotemporal control of this highly versatile and widely implemented coupling reaction, perhaps the most straightforward route is to trigger the reaction by utilizing a photocaged amine (PCA).11–14 This spatiotemporal control can allow the on-demand initiation of a specific reaction, which is highly desirable under certain circumstances, and broaden its applications for the synthesis of functional materials. Due to the advantages of light-emitting diodes (LEDs), including lower costs, better environment-friendliness and larger application areas, the photochemical reaction with LEDs has demonstrated enormous potential as a substitute for the traditional Hg lamp-induced reaction;15 however, classical PCAs usually suffer from poor light absorption properties for λ ≥ 365 nm because they were initially developed specifically for UV Hg lamps. Hence, a major challenge with most PCAs is that their relatively low quantum yields easily leads to low photosensitivity, especially under the radiation of LEDs.
In this context, the phototriggered base proliferation (PBP) reaction is expected to play a significant role in the thiol-Michael addition reaction to improve the photo-initiated efficiency. The PBP reaction, which involved an autocatalytic decomposition of a base amplifier (BA) triggered by an amine catalyst from PCA, was pioneered by Ichimura and Arimitsu in 2000.16 The geometric progression of proliferated amine species causes a dramatic increase in the catalytic concentration and thus accelerates base-sensitive reactions. PBPs have been designed and applied in various types of photoreactive materials.17–20 Compared to PCA systems, this nonlinear organic reaction displays several hallmarks, such as high sensitivity, high quantum yield and short reaction times. By optimizing this unique proliferation characteristic, PBP reaction is transformed into a powerful and versatile methodology for nucleophile-initiated thiol-Michael addition photoclick reaction.
Experiments
Materials
2-(2-Nitrophenyl)propyl chloroformate (NPPOC-Cl, 95%, Sigma-Aldrich), 9-fluorenylmethyl chloroformate (Fmoc-Cl, 98%, Shanghai Darui Finechem), hexylamine (HA, 98%, Aladdin-reagent), β-mercaptoethanol (ME, >98%, Jian Yang Biotechnology), ethyl mercaptoacetate (EM, 98%, Aladdin-reagent), hexanethiol (HE, 96%, Aladdin-reagent), thioglycolic acid (TA, ≥98%, Aladdin-reagent), benzyl mercaptan (BE, 99%, Alfa Aesar), acrylic acid (AA, Tianjin Fu Chen Chemical Reagent Factory), and butyl acrylate (BA, 99%, Aladdin-reagent) were used as received. All other chemicals used were of analytical grade and used without further purification.
Methods
The NMR spectra were obtained on a Varian 400 MHz spectrometer with DMSO-d6 and TMS as the solvent and internal standard, respectively. FTIR spectra were obtained over the wavenumber range of 400–4000 cm−1 on a Thermo Nicolet/Nexus 670 spectrophotometer and recorded from 32 scans with a resolution of 4 cm−1. Real-time infrared spectroscopy (RTIR) was conducted to monitor the thiol–ene Michael addition reaction kinetics using a modified Nicolet 6700 (ThermoElectron, USA). UV-vis absorption spectra were obtained on a Perkin Elmer Lambda 750 UV-visible spectrophotometer. Electrospray ionization mass spectra (ESI-MS) were acquired on a Thermo Finnigan LCQ DECA XP ion trap mass spectrometer, equipped with an ESI source.
A UV-LED source (UVEC-4II, Lamplic Technology China) with a 365 nm emission wavelength was used for the photochemical reaction of the NPPOC-HA and PBP system with an irradiation intensity of 50 mW cm−2 (monitored by a radiometer, type UV-A, Photoelectric Instrument Factory, Beijing Normal University).
The resulting mixtures were uniformly smeared onto KBr crystals in a horizontal transmission apparatus, and then the reactions were initiated by the UV-LED source. Thiol conversion was monitored with the S–H absorption peak at 2570 cm−1, and ene conversion was monitored with the C
C absorption peak at 810 cm−1. Conversions were calculated by comparing the ratio of peak areas after the reaction to the peak areas prior to the reaction.
Synthetic procedures and characterization
Preparation of 2-(2-nitrophenyl)propyloxycarbonyl-hexylamine (NPPOC-HA). 2-(2-Nitrophenyl)propyl chloroformate (4 mmol, 0.9746 g) was dissolved in CH2Cl2 (40 mL), and then added dropwise to a solution of hexylamine (8.4 mmol, 0.8499 g) in CH2Cl2 (20 mL). After that, the mixture was stirred under cooling for 20 min and at room temperature for 5 hours. Finally, the solution was washed thrice successively with water, a 5% HCl aqueous solution, and a saturated NaCl solution, dried over anhydrous MgSO4, and evaporated to obtain a light yellow liquid; then, the crude product was further purified by silica gel column chromatography using hexane
:
EtOAc (10
:
1) to obtain a pure light yellow oil as the product. Yield: 76%. 1H NMR (400 MHz, CDCl3, δ): 7.65 (d, 1H, H-a), 7.49 (t, 1H, H-b), 7.40 (d, 1H, H-c), 7.28 (t, 1H, H-d), 4.01–4.16 (m, 2H, H-e), 3.63 (t, 1H, H-f), 1.19–1.36 (t, 13H, H-g-i), 0.80 (t, 3H, H-h). 13CNMR (400 MHz, CDCl3, δ): 156.09, 150.77, 137.45, 132.52, 128.25, 127.25, 123.95, 68.57, 67.21, 44.35, 41.60, 40.98, 33.25, 31.42, 29.82, 28.79, 26.32, 26.03, 22.51, 17.78, 17.32, 13.98.
Preparation of 9-fluorenylmethoxycarbonyl-hexylamine (Fmoc-HA). Hexylamine (15.4 mmol, 1.561 g) in CH2Cl2 (20 mL) was slowly added to an ice cooled solution of Fmoc-Cl (7.72 mmol, 2.015 g) in CH2Cl2 (50 mL), and then the mixture was stirred under cooling for 20 min and at room temperature for 1.5 hours. The solution was washed thrice successively with water, a 5% HCl aqueous solution, and a saturated NaCl solution, dried over anhydrous MgSO4, and evaporated to obtain a solid; then, the solid was recrystallized from ethanol to obtain a white crystalline. Yield: 87%. 1H NMR (400 MHz, CDCl3, δ): 7.77 (d, 2H, H-a), 7.60 (d, 2H, H-b), 7.40 (t, 2H, H-c), 7.31 (t, 2H, H-d), 4.41 (d, 2H, H-e), 4.22 (t, 1H, H-f), 3.19 (m, 2H, H-g), 1.30 (m, 8H, H-h), 0.89 (t, 3H, H-i). 13CNMR (400 MHz, CDCl3, δ): 156.10, 144.56, 142.01, 128.81, 128.40, 126.78, 120.74, 66.98, 47.52, 42.67, 31.71, 29.72, 26.08, 22.80, 13.98.
Base proliferation in solution
n-Hexylamine (5 mmol L−1) was added to a DMSO-d6 solution of Fmoc-HA (100 mmol L−1) in an NMR tube. The tube was sealed and subjected to real-time NMR measurement at 25 °C. The proton of the methylene group was used to monitor the consumption of the Fmoc-HA.
Results and discussion
To develop the highly effective phototriggered base proliferation system, the highly active implementation presented in this paper has taken the following points into consideration. First, the photolytic deprotection reaction of the PCA should not generate any free radicals; the radical-mediated homopolymerization will reduce the ultimate conversion and thus affect the system's orthogonality and “click” nature. Moreover, thiols absorb light much more readily at lower wavelengths (<320 nm), and thus PCA should have relatively strong absorption above 320 nm. In this paper, 2-(2-nitrophenyl)propyloxycarbonyl-hexylamine (NPPOC-HA) was synthesized by choosing the photolabile protecting group of 2-nitrobenzyl. Second, it should be noted that primary amines are more effective for the thiol-Michael addition reaction than secondary amines.6 Beside the possessing lower volatility, the liberated amine from base amplifier should be strong enough to catalyze subsequent base proliferation reactions. Generally, the higher pKa of the proliferated amine resulted in a faster proliferation rate. Consequently, we designed 1-(9-fluorenylmethoxycarbonyl)-4-hexylamine (Fmoc-HA) by utilizing the ideal n-hexylamine (HA, pKa = 10.56). The results from 1H NMR, 13C NMR and FTIR revealed that the targeted NPPOC-HA and Fmoc-HA were successfully obtained.
The phototriggered base proliferation initiated thiol-Michael addition reaction is described in Fig. 1, in which the regenerated n-hexylamine that is liberated from UV-irradiated NPPOC-HA and used as a phototrigger can promptly actuate highly effective base proliferation reactions; copious n-hexylamine species are thus generated such as in a domino reaction. Even under LED irradiation at a localized region, the regenerated n-hexylamine from NPPOC-HA can also diffuse into unirradiated regions and effectively actuate base proliferation reactions in three-dimensional spaces. The abruptly proliferated n-hexylamine molecules can be utilized to catalyze the thiol-Michael addition reaction, and this potentially results in enhanced photosensitivity and fast reaction rates. As a phototrigger, NPPOC-HA exhibits good absorption characteristics with a maximum at 198 nm and a tail above 300 nm. It is obvious that NPPOC-HA has poor absorption, but it can also be used for UV (>320 nm) irradiation reactions. In particular, the extinction coefficient at 365 nm is 782 M−1 cm−1.
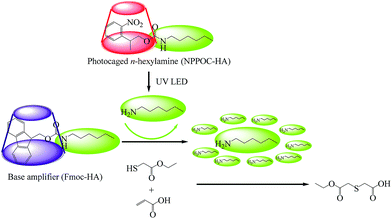 |
| Fig. 1 Schematic illustration of phototriggered base proliferation initiated thiol-Michael addition reaction. | |
ESI-MS was employed to detect the photodecomposed basic compounds included photogenerated n-hexylamine and proliferated n-hexylamine from 365 nm LED radiated PBP in DMSO. Before irradiation (Fig. 2a), signal molecules of n-hexylamine were not obtained in PBP solution. As shown in Fig. 2b, a tiny amount of protonated n-hexylamine (m/z = 102.3) was detected by ESI-MS after 30 min irradiation, which was direct evidence for the generation of n-hexylamine from irradiated NPPOC-HA. Furthermore, the protonated n-hexylamine (m/z = 102.3) was also successfully detected (Fig. 2c), and it is worth noting that the signal intensity of n-hexylamine+ in Fig. 2b is apparently smaller than Fig. 2c, as expected. These facts suggest that the highly effective base proliferation reaction can be readily actuated by employing NPPOC-HA as a phototrigger, and the massive n-hexylamine species can be further used to catalyze subsequent chemical reactions.
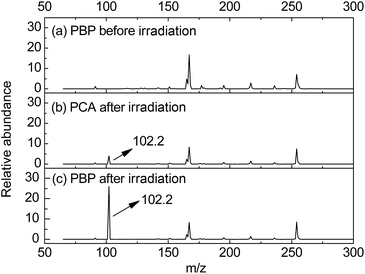 |
| Fig. 2 Positive ion ESI-MS spectra (a) PBP before, (b) PCA after, and (c) PBP after 365 nm LED irradiation for 30 min in DMSO. Experimental conditions: PCA, [NPPOC-HA] = 0.01 mM; PBP, [NPPOC-HA] = 0.01 mM, [Fmoc-HA] = 0.02 mM. | |
Prior to evaluating the PBP-initiated thiol-Michael addition photoclick reaction, base proliferation in solution was recorded by real-time 1H NMR because the ability to initiate a rapid thiol-Michael addition reaction is primarily dependent on the base proliferation rate. As shown in Fig. 3(1), there was no proton signal (Hb, 6.25 ppm) of vinyl found before the addition of hexylamine (Fig. 3(1)a), once the amine was added into the solution (Fig. 3(1)b), the proton signal (Hb, 6.25 ppm) of vinyl gradually increased as the reaction proceeded (Fig. 3(1)c). By monitoring the disappearing proton signals (Ha, 4.23 ppm) of methylene and the DMSO-d6 solvent peak at 2.5 ppm as an internal standard based on 1H NMR, and integrating the corresponding proton peak, the conversion curve in Fig. 3(1) was obtained. The approximately sigmoidal curve indicates that the decomposition of Fmoc-HA autocatalytically proceeds and lead to the proliferation of n-hexylamine at 25 °C, and almost 70% conversion was obtained after 40 min. Astonishingly, when the reaction temperature was increased to 80 °C, the reaction profile was too fast to be measured by real-NMR, which indicated a complete reaction occurred in <2 min.
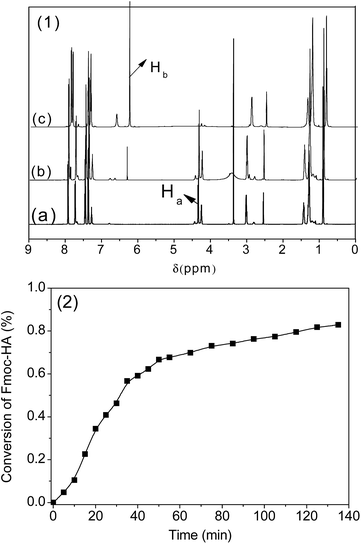 |
| Fig. 3 (1) 1H NMR spectral changes of Fmoc-HA in DMSO-d6 (100 mmol L−1) (a) in the absence of and (b) in the presence of n-hexylamine (5 mmol L−1) at 25 °C for 2 min and (c) for 120 min; (2) the conversion of Fmoc-HA (100 mmol L−1) as a function of time in the presence of n-hexylamine (5 mmol L−1) in DMSO-d6 at 25 °C. | |
Next, real-time Fourier transform infrared (FTIR) spectroscopy was used to compare the catalytic behavior of PCA and PBP systems upon 365 nm LED irradiation at room temperature to demonstrate the potential of the two systems as photocatalysts for the thiol-Michael addition reaction; ethyl mercaptoacetate and acrylic acid were selected as model substrates. As shown in Fig. 4, these reactions could be rapidly actuated under photo-irradiation without the presence of obvious induction periods, and the results of both PCA and PBP systems showed near complete conversions of both thiol and acrylate in 8 min. As expected, under the same irradiation conditions, the system with a higher amine loading exhibited a faster reaction rate. In the case of the PCA system, an 80% thiol conversion required 3.44 min, and the maximum initiation rate (Rpmax) was 49.4 min−1. Astonishingly, for the PBP system, the addition of Fmoc-HA led to a sharp decrease of Tc80% from 3.44 min to 2.38 min and a slight increase of Rpmax from 49.4 min−1 to 57.6 min−1; 80% thiol conversion was obtained in 1.21 min. Moreover, the PBP reaction without irradiation does not have a noticeable effect on the final conversion of thiol-Michael reaction. The faster photoefficiency of the PBP system can be attributed to the massive increase in the amount of amine catalysts, and this dramatically enhanced the reaction rate.
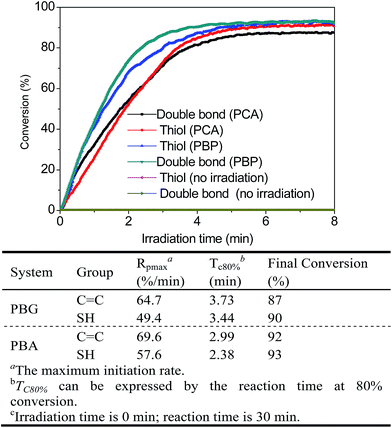 |
| Fig. 4 Conversion vs. time for the reaction for stoichiometric acrylic acid/ethyl mercaptoacetate mixtures upon irradiation by a 365 nm LED: (a) PCA system, [NPPOC-HA] = 0.16 mmol, (b) PBP system, [NPPOC-HA] : [Fmoc-HA] = 1 : 1.5, ethyl mercaptoacetate (2 mmol), acrylic acid (2 mmol) and butyl acrylate (2 mmol). | |
The adaptation of different monothiols catalyzed with PBP under the same 365 nm LED light source was critical in designing a highly effective base-catalyzed thiol-Michael addition photo-click reaction. In light of this, all of the monothiols in Fig. 5 were evaluated. Closer analysis of the data indicates that all monothiols obtained >90% conversation, demonstrating that PBP can be utilized as a highly effective photo-trigger for the base-catalyzed thiol-Michael photo-click reaction.
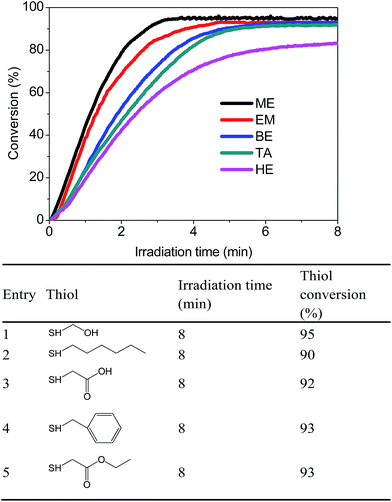 |
| Fig. 5 Conversion vs. time for the reaction with different monothiols and acrylic acid catalyzed with the PBP system upon irradiation by a 365 nm LED: (a) PCA system, [NPPOC-HA] = 0.16 mmol, (b) PBP system, [NPPOC-HA] : [Fmoc-HA] = 1 : 1.5, monothiols (2 mmol), acrylic acid (2 mmol) and butyl acrylate (2 mmol). | |
To further investigate the properties of the propagating reaction, we will address the need for the kinetic characterization of the PBP reaction by characterizing one of its distinguishing features: the long life-times of the active centers. Once the thiol-Michael addition reaction is initiated by the 365 LED light source, it continues after the light is shut off. As shown in Fig. 6, the sample illuminated for only 1 min continues to react for more than 11 min in the dark, and its final conversion rises to ∼77% from a threshold value of ∼40%. The same final conversion value required a sustained illumination of 2.74 min. This implies that enough active centers are produced even after an illumination time as low as 1 min; this is significant for the photo-screened reactions.
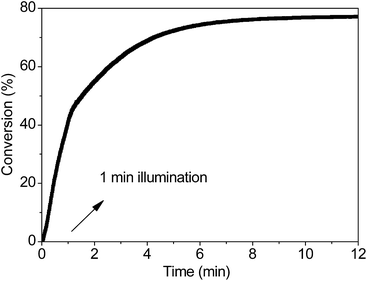 |
| Fig. 6 Phototriggered propagating reaction after initial first 1 min illumination by 365 LED light source. Experimental conditions: [NPPOC-HA] : [Fmoc-HA] = 1 : 1.5, [NPPOC-HA] = 0.16 mmol, β-mercaptoethanol (2 mmol), ethyl mercaptoacetate (2 mmol), acrylic acid (2 mmol) and butyl acrylate (2 mmol). | |
Conclusions
In conclusion, we have described a detailed study on the phototriggered base proliferation-induced thiol-Michael addition photo-click reaction as a powerful 365 nm LED photoclick tool. The results suggest that PBP offers a substantial breakthrough in the photoefficiency of amine generation, which results in a marked improvement in photosensitivity. Practically, this system was successfully used for the photo-screened reactions.
Acknowledgements
This research was financially supported by National Natural Science Foundation of China (21404042), Natural Science Foundation of Guangdong Province (2014A030310166), International Postdoctoral Exchange Fellowship Program ([2014] 29), Scientific Research Project of Shenzhen Polytechnic (601522k24011) and Fundamental Research Funds for the Central Universities (2016ZM060).
Notes and references
- H. C. Kolb, M. G. Finn and K. B. Sharpless, Angew. Chem., Int. Ed., 2001, 40, 2004 CrossRef CAS PubMed.
- J. E. Moses and A. D. Moorhouse, Chem. Soc. Rev., 2007, 36, 1249 RSC.
- C. E. Hoyle and C. N. Bowman, Angew. Chem., Int. Ed., 2010, 49, 1540 CrossRef CAS PubMed.
- J. W. Chan, C. E. Hoyle, A. B. Lowe and C. N. Bowman, Macromolecules, 2010, 43, 6381 CrossRef CAS.
- G. Z. Li, R. K. Randev, A. H. Soeriyadi, G. Rees, C. Boyer, Z. Tong, T. P. Davis, C. R. Becer and D. M. Haddleton, Polym. Chem., 2010, 1, 1196 RSC.
- J. W. Chan, H. Wei, H. Zhou and C. E. Hoyle, Eur. Polym. J., 2009, 45, 2717 CrossRef CAS.
- O. Altintas, A. P. Vogt, C. Barner-Kowollik and U. Tunca, Polym. Chem., 2012, 3, 34 RSC.
- D. P. Nair, N. B. Cramer, J. C. Gaipa, M. K. McBride, E. M. Matherly, R. R. McLeod, R. Shandas and C. N. Bowman, Adv. Funct. Mater., 2012, 22, 1502 CrossRef CAS.
- L. Maleki, U. Edlund and A. C. Albertsson, Biomacromolecules, 2015, 16, 667 CrossRef CAS PubMed.
- K. Jin, N. Wilmot, W. H. Heath and J. M. Torkelson, Macromolecules, 2016, 49, 4115 CrossRef CAS.
- W. Xi, M. Krieger, C. J. Kloxin and C. N. Bowman, Chem. Commun., 2013, 49, 4504 RSC.
- S. Chatani, R. J. Sheridan, M. Podgórski, D. P. Nair and C. N. Bowman, Chem. Mater., 2013, 25, 3897 CrossRef CAS.
- W. Xi, H. Peng, A. Aguirre-Soto, C. J. Kloxin, J. W. Stansbury and C. N. Bowman, Macromolecules, 2014, 47, 6159 CrossRef CAS PubMed.
- X. Zhang, W. Xi, C. Wang, M. Podgórski and C. N. Bowman, ACS Macro Lett., 2016, 5, 229 CrossRef CAS PubMed.
- P. Xiao, J. Zhang, F. Dumur, M. A. Tehfe, F. Morlet-Savary, B. Graff, D. Gigmesb, J. P. Fouassierc and J. Lalevée, Prog. Polym. Sci., 2015, 41, 32 CrossRef CAS.
- K. Arimitsu, M. Miyamoto and K. Ichimura, Angew. Chem., Int. Ed., 2000, 39, 3425 CrossRef CAS PubMed.
- H. Mohapatra, K. M. Schmid and S. T. Phillips, Chem. Commun., 2012, 48, 3018 RSC.
- T. Sasaki and H. Yaguchi, J. Polym. Sci., Part A: Polym. Chem., 2009, 47, 602 CrossRef CAS.
-
(a) A. Igarashi, K. Arimitsu, T. Seki and K. Ichimura, J. Mater. Chem., 2008, 18, 560 RSC;
(b) M. Furutani, H. Kobayashi, T. Gunji, Y. Abe and K. Arimitsu, J. Polym. Sci., Part A: Polym. Chem., 2015, 53, 1205 CrossRef CAS;
(c) K. Arimitsu, K. Tomota, S. Fuse, K. Kudo and M. Furutani, RSC Adv., 2016, 6, 38388 RSC;
(d) K. Arimitsu, Y. Kumazawa and M. Furutani, J. Polym. Sci., Part A: Polym. Chem., 2015, 53, 2440 CrossRef CAS;
(e) K. Arimitsu, S. Amano and M. Furutani, RSC Adv., 2016, 6, 7893 RSC.
-
(a) M. He, X. Huang, Z. Zeng and J. Yang, Macromolecules, 2013, 46, 6402 CrossRef CAS;
(b) M. He, S. Jiang, R. Xu, J. Yang, Z. Zeng and G. Chen, J. Polym. Sci., Part A: Polym. Chem., 2014, 52, 1560 CrossRef CAS.
Footnote |
† These authors contributed equally to this work. |
|
This journal is © The Royal Society of Chemistry 2017 |
Click here to see how this site uses Cookies. View our privacy policy here.