DOI:
10.1039/C6RA27980E
(Paper)
RSC Adv., 2017,
7, 20469-20479
Biocompatible terpolyesters containing polyhydroxyalkanoate and sebacic acid structural segments – synthesis and characterization†
Received
9th December 2016
, Accepted 29th March 2017
First published on 10th April 2017
Abstract
A convenient synthetic route of poly(3HB-co-3HH-co-SEB) terpolyesters has been reported. The developed protocol consists of two steps; poly(3-hydroxybutyrate-co-3-hydroxyhexanoate) (PHBHx) oligoesterdiols were synthesized via selective reduction of biopolyesters and then via their polycondensation with sebacoyl chloride the biodegradable terpolyesters, poly(3HB-co-3HH-co-SEB), were obtained. The structure of the obtained terpolyesters was established by NMR analysis supported by mass spectrometry. Modification of poly(3-hydroxybutyrate-co-3-hydroxyhexanoate) by incorporating units derived from sebacic acid into its chains resulted in terpolyesters with better physico-chemical properties than the starting PHBHx biopolyester. These new polyester materials have greater thermal stability than the starting biopolyester. Furthermore, the introduction of sebacic acid units to a polyester chain leads to the reduction of the glass transition temperatures of the materials and reduces the required processing temperature. A preliminary study confirmed that poly(3HB-co-3HH-co-SEB) terpolyesters can be used to create scaffolds for cell cultures in the form of a three-dimensional spatial structure. Moreover, a toxicity test of the obtained terpolyester carried out on human cells demonstrated that the novel terpolyester is not toxic to human cells in vitro.
Introduction
Polyhydroxyalkanoates (PHAs) are naturally produced polyesters that are accumulated as a carbon storage material by a large number of bacteria, usually under conditions of limited nutrients (such as ammonium, sulfate, and phosphate) and an excess supply of a carbon source.1–3 PHAs are based on a number of different hydroxyacid monomers, which differ in the length of the alkyl side chain in the beta-position. PHAs also include poly(3-hydroxybutyrate) and its copolymers, which are more interesting, because it is possible to change the copolymer's physical and thermal properties by varying the molecular structure, average co-monomer composition, and co-monomer composition distribution.4 Aliphatic biopolyesters have a leading position among the biodegradable polymers, because their hydrolytic and/or enzymatic degradation yields hydroxyacids that in most cases are metabolized. Potential uses of PHAs in the medical field are anticipated in wound treatment (sutures, skin substitutes, surgical meshes, staples), vascular system applications (heart valves, pericardial patches) orthopedics (scaffolds for cartilage engineering, spinal cages, bone graft substitutes), and drug delivery system.5–8 The application of polymers for medical devices and tissue engineering requires appropriate biocompatibility, as well as mechanical and degradation properties of the material. Regardless of the variety of PHAs copolymers available on the world market, until now no biodegradable polymer could fulfill all of the expected requirements needed for wide medical applications. Some modifications of the PHAs can be introduced directly biotechnologically by changing the structure of the alkyl side chains in the beta position. The most promising direction for the development of PHA on the biosynthesis level is the production a group of medium chain length PHA (mcl-PHA). The copolyesters of this group are characterized by relatively low glass transition temperatures (Tg), melting point (Tm) and low degree of crystallinity. However, the continuous elongation of the side chain in the repeating units leads to too large reduction in Tm value which results in increase of the stickiness/tackiness and thus restricting its use as a structural material.9 However, PHA biopolyesters containing longer aliphatic chains in the main chain are not well known. The only exception is a copolymer 3-hydroxybutyric acid with 4-hydroxybutyric acid, P(3HB4HB), which has much better physicochemical properties than typical PHA biopolyesters.10,11 Therefore, a regular investigation has recently been conducted on the development of new polymeric biomaterials with certain properties, which are based on matrices or on structural segments derived from PHA biopolyesters.8,12,13 One of the possible ways to use PHA biopolyesters in the synthesis of tailored biomaterials is to obtain from them some oligomers that can be used in further synthesis of new polymeric materials. The oligomers from PHAs can be obtained through controlled chemical degradation of the high-molar mass PHAs. One type of chemical degradation is a partial saponification by KOH/18-crown-6 system or an aqueous solution of tert-butylammonium hydroxide, which can be carried out in a CHCl3/H2O system. This method allows to obtain macroinitiators that contain carboxylate and unsaturated end groups.14–16 Macroinitiators containing the same end groups can also be prepared using a thermal method that is based on the random polymer-chain scission mechanism via intramolecular stereoselective cis-elimination17 or via E1cB degradation.18–20 The other type of controlled chemical degradation is alcoholysis under acid conditions; the alcohols that can be used for this process are: methanol,21,22 glycol or glycerol.23 In the case of controlled degradation by ethylene glycol or glycerol, obtained oligomers are terminated with several hydroxyl groups.22 An alternative potential synthesis method for oligoesterdiols is the reduction of polyesters. Our recently published results indicated that PHA biopolyesters can constitute a source of valuable reactants (e.g., reactive oligodiols of PHA) which can be useful for the synthesis of new polymeric materials.24 The macromonomers obtained with two hydroxyl end groups (oligodiols) are very useful in the preparation of a wide spectrum of polyesters or polyurethanes.
The purpose of the present study was to develop biodegradable and biocompatible materials with tailor-made properties that enable their use as a prospective material for the preparation of three-dimensional cell culture scaffolds for application in regenerative medicine. The polymeric materials used for these purpose should comply with the following requirements. Three dimensional scaffolds should have a large expansion surface area and a large open pore volume ratio that allows them to be populated with a maximum number of cells. On the other hand, such scaffolds should have satisfactory mechanical properties that ensure dimensional stability during cell culture and surgical procedures as well as assure post-implantation time needed to reconstruct the tissue specific to the site of the defect. An effective method for the synthesis of new polymeric materials was developed using poly(3-hydroxybutyrate-co-3-hydroxyhexanoate), (PHBHx) oligodiols via their polycondensation with sebacoyl chloride. Great emphasis was focused on the determination of correlation between the chemical structure of the obtained materials and their properties in order to receive a variety of materials suitable for regenerative medicine.
Experimental section
Materials
Poly(3-hydroxybutyrate-co-3-hydroxyhexanoate), PHBHx, was provided by the Procter and Gamble Company (Cincinnati, USA). The sample of PHBHx was characterized by gel permeation chromatography (GPC) and 1H and 13C NMR. The number-average molar mass of PHBHx, determined by GPC, was Mn = 700
000 g mol−1 with dispersity Mw/Mn = 3.5. The composition of PHBHx, estimated by 1H NMR, was 88 mol% of HB units and 12 mol% of HH units.
Lithium Borohydride (LiBH4) (2.0 M solution in THF) (Aldrich), hydrochloric acid (HCl) (35–38% pure P.A. – basic, Avantor Gliwice), chloroform (CHCl3) (98.5% pure P.A. – basic Avantor Gliwice), sebacoyl chloride (99%, Aldrich), tetrahydrofuran (THF) (pure, Avantor Gliwice) were distilled over potassium–sodium alloy just before using.
HEK293 human embryonic kidney cells and WI-38 normal human lung fibroblasts were obtained from the American Type Culture Collection (ATCC).
Methods
Preparation of oligo-diols. PHA oligodiols were obtained via the selective partial reduction of the ester bonds of a PHBHx biopolyester using lithium borohydride (LiBH4).
General procedure. A total of 1 g (0.004 mmol) of PHBHx was introduced into a dried three-necked flask that was equipped with a thermometer, a reflux condenser, and a dropping funnel. Anhydrous tetrahydrofuran (THF) (100 cm3) was added, and the mixture was stirred under argon until complete solubilisation of the polyester was achieved. The polymer solution was subsequently cooled using an ice/water bath to a temperature less than 20 °C, and 1.5 cm3 of the 2.0 M solution of lithium borohydride in THF plus 10 cm3 of anhydrous THF were then added dropwise. The LiBH4 solution was added dropwise over 15 minutes. The reaction mixture was then stirred for 4 hours, 100 cm3 of a 1% HCl water solution was added dropwise, and the reaction mixture was stirred for 30 min. Subsequently, the reaction mixture was transferred to a separator, 100 cm3 of chloroform was added, and the organic layer was washed several times with distilled water. The chloroform fraction, which contained oligodiols, was evaporated under vacuum to obtain the product. The obtained PHA oligodiols were subjected to GPC, 1H NMR, and ESI-MSn analyses.
Polycondensation of PHA oligodiols with sebacoyl chloride. Polycondensation process of PHA oligodiols with sebacoyl chloride was carried out in the melt. Before polycondensation process, the oligodiols obtained via reduction of PHBHx were dried by distillation from toluene solution. The molar ratio of PHBHx oligodiols and sebacoyl chloride in this process was 1
:
1. Dried oligodiols were melted at 120 °C and then the sebacoyl chloride was added in three equal parts, the first one was added at the beginning of the process and the next ones after one and two hours. The process was carried out under a reduced pressure of 600 mmHg for 4 hours at a constant temperature of 120 °C. After 4 hours, the reaction mixture was dissolved in chloroform and transferred to a separatory funnel and then washed twice with distilled water. The organic layer was then precipitated into hexane (ratio of chloroform: hexane 1
:
10 v/v) and then dried under vacuum and characterized using analytical techniques such as 13C NMR and GPC.
Preparation of polymer film on coverslips. Polymer films on coverslips were prepared for biological studies. The obtained terpolyester poly(3HB-co-3HH-co-SEB) was dissolved in 1,1,1,3,3,3-hexafluoro-2-propanol (1 g per 10 cm3) and then the solutions were transferred by Pasteur pipette on microscope cover glasses (diameter 13 mm, VWR International). The prepared coverslips were dried under vacuum (10 mmHg) for 24 hours.
Assessment of toxicity to human cells. Polymer coated coverslips were sterilised by immersion in 100% ethanol and allowed to air dry before being placed in 24-well tissue-culture treated plates (Corning, Sigma-Aldrich). 10000 HEK293 human embryonic kidney cells or 10000 WI-38 normal human lung fibroblasts were plated into either empty wells or wells containing coated coverslips. Cells were maintained in DMEM medium (Lonza, Slough, UK) supplemented with 10% foetal calf serum, 50
units mL−1 penicillin and 50 μg mL−1 streptomycin (Lonza, Slough, UK) for 5 days.After 5 days of growth, cell viability was assessed by standard MTT assay. Cells were incubated in growth media containing 5 mg mL−1 MTT (Sigma-Aldrich) for 2 h at 37 °C, followed by solubilization of the resulting insoluble formazan crystals with 180 μL of dimethyl sulfoxide and 20 μL of Sorensen's glycine buffer. Absorbance at 540 nm was read using a microplate reader (Multiskan™ Thermofisher scientifics, USA). To ensure the polymer alone did not generate false positive results, polymer-coated plates were incubated in growth media without the addition of cells and subjected to MTT assay. The resulting absorbance measurements were subtracted form those obtained from cell-coated coverslips. Each experiment was carried out four times to generate average viability values.
To confirm the MTT assay results, cells were observed by phase-contrast microscopy at 100× magnification.
Three-dimensional scaffolds preparation. In this study, the initial attempt was also to produce three-dimensional scaffolds for cell cultures derived from obtained terpolyester poly(3HB-co-3HH-co-SEB). To produce this kind of three-dimensional scaffolds, obtained terpolyester in an amount of 0.3 g was mixed with 2.7 g of sodium chloride, to the mixture was added 0.5 cm3 of methylene chloride and then a roller, having a diameter of 0.5 cm in a prepared form, was formed. After 3 h the formed roller was removed from the form and placed in a vacuum oven for 24 h to remove residual methylene chloride. The next step was placing the molded roller in a dish with distilled water to elute the sodium chloride. Distilled water in the initial step (8 h) elution was replaced every 2 h and every 24 h for 7 days. After a period of 7 days, the obtained substrate was dried in a vacuum oven for 3 days.
GPC analysis. The number-average molar mass (Mn) and the molecular mass distribution index (Mw/Mn) of the plain PHA samples were determined by GPC that was conducted in a CHCl3 solution at 35 °C with a flow rate of 1 mL min−1 using a Spectra-Physic 8800 solvent delivery system. This system had a set of two PLgel 5 μm MIXED-C ultra-high efficiency columns and a Shodex SE 61 refractive index detector. For the GPC analysis of the PHA oligodiols, a PLgel 3 μm mixed-E column and THF, as the solvent, were used. A sample solution volume of 10 μL (concentration of 1% w/v) was injected. Polystyrene standards with a narrow molecular mass distribution were used to generate calibration curves.
NMR analysis. The 1H NMR spectra were recorded using a Bruker-Avance II 600 MHz with Ultrashield Plus Magnets. The 1H spectra were run with CDCl3 as the solvent and using tetramethylsilane (TMS) as an internal standard. The 13C NMR spectra were recorded on a Bruker-Avance II 150 MHz, using CDCl3 and TMS. 1H NMR spectra were obtained with 64 scans, an 11 ls pulse width and 2.65 s acquisition time, whereas 13C NMR spectra were obtained with 20
480 scans, a 9.40 ls pulse width, and 0.9088 s acquisition time.
ESI-MSn experiments. Electrospray mass spectrometry analysis was performed using a Finnigan LCQ ion trap mass spectrometer (Finnigan, San Jose, CA, USA). The polyester samples were dissolved in a chloroform/methanol system (1
:
1 v/v), and the solutions were introduced into the ESI source by continuous infusion using the instrument's syringe pump at a rate of 5 μL min−1. The LCQ ESI source was operated at 4.5 kV, and the capillary heater was set to 200 °C. Nitrogen was used as the nebulising gas. For ESI-MS/MS experiments, the ions of interest were isolated monoisotopically in the ion trap and were collisionally activated. The helium damping gas that was present in the mass analyser acted as a collision gas. The RF amplitude, which had a significant voltage range, was set to a value that caused the peak height of the molecular ion to decrease by at least 50%. The analysis was performed in the positive-ion mode.
DSC analysis. Differential scanning calorimetry (DSC) measurements were performed with a TA DSC 2010 apparatus (TA Instruments, New Castle, DE) from −50 °C to 200 °C, at heating rates of 10 °C min−1. All of the experiments were performed under a nitrogen atmosphere (flow = 50 mL min−1). The instrument was calibrated with indium and gallium standards. The melting temperature (Tm) and the enthalpy value were obtained from the first heating run.
TGA analysis. Thermogravimetric analysis (TGA) measurements were performed with a TGA/DSC1 apparatus (Mettler-Toledo) from 25 °C to 400 °C, at heating rates of 10 °C min−1. All of the experiments were performed under a nitrogen atmosphere (flow = 60 mL min−1).
Scanning electron microscopy (SEM). The surface morphology of obtained scaffolds was observed using a scanning electron microscopy Nanolab 7 (Semco). The observations were performed at an accelerating voltage of 15 kV, on samples sputter-coated with gold.
Results and discussion
A series of new polyesters was obtained by polycondensation reaction of PHA oligodiols with sebacic acid derivatives, such as acid dichlorides. Selection of sebacic acid and its derivatives as reagents for the polycondensation were dictated by the fact that sebacic acid is a natural metabolic intermediate in the oxidation of medium- to long-chain fatty acids.25–27 Recently, sebacic acid has been widely used for the preparation of the poly(glycerol-sebacate) (PGS) copolymers by its polycondensation reaction with glycerol. PGS may be useful in medical application, especially for tissue engineering, drug delivery systems and bioresorbable sutures.28 Such strategies should offer the possibility to synthesize polymeric materials based on structural units derived from PHA biopolyesters with greater potential application in medicine.
The controlled selective reductive degradation of PHB an P(3HB-4HB) biopolyesters with the aid of lithium borohydride were described by our group recently.23 The elaborated method allows to obtain from those biopolyesters oligodiols with controlled chemical structure and molecular weight. In this studies we decided to use this method for obtain oligodiols from PHBHx biopolyester which belong to mcl-PHA group.
The first step in this study was focused on the optimization of developed method in order to find the reaction conditions under which the reduction of PHAs (containing structural units with the longer aliphatic side chains mcl-PHAs) can be carried out, according to the Scheme 1.
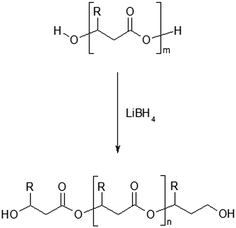 |
| Scheme 1 Reduction reaction of poly(3-hydroxybutyrate-co-3-hydroxyhexanoate) by lithium borohydride (R = CH3 for 3HB units or R = C3H7 for 3HH units). | |
A series of oligodiols were obtained via the selective controlled reductive cleavage of high molecular weight PHBHx biopolyester using lithium borohydride. The average molar masses of the PHBHx oligodiols were controlled by the amount of reduction agent. PHBHx oligodiols with an average molar mass range of approximately 500–5000 g mol−1 were synthesised, and their characterisation is summarised in Table 1.
Table 1 PHAs starting samples and PHAs oligodiols obtained from them
Entry no. |
PHBHx |
Amount of LiBH4 (mmol) |
PHBHx-diols |
Mna (g mol−1) |
Mw/Mna |
Content of 3-HH unitsb (mol%) |
Mna (g mol−1) |
Mw/Mna |
Content of 3-HH unitsb (mol%) |
Estimated by GPC in chloroform solution at 35 °C using polystyrene standards. Composition of starting PHBHx and PHBHx oligodiols estimated from 1H NMR. |
1 |
700 000 |
3.5 |
12.5 |
3 |
1200 |
2.0 |
12.3 |
2 |
2.4 |
2000 |
1.9 |
12.0 |
3 |
2.0 |
3500 |
2.5 |
12.6 |
Structural characterization of the PHBHx oligodiols by 1H NMR analysis
The 1H NMR spectra of the starting high molar mass poly(3-hydroxybutyrate-co-3-hydroxyhexanoate) biopolyester and the oligomers derived from it, which was obtained via the selective reduction, are presented in Fig. 1 and 2, respectively. The signals present in the 1H NMR spectrum (Fig. 1) of the high molar mass poly(3-hydroxybutyrate-co-3-hydroxyhexanoate) biopolyester correspond with the protons of the 3-hydroxybutyrate repeating units (labelled 1, 2 and 3) and 3-hydroxyhexanoate repeating units (signals labelled 4, 5, 6, 7 and 8). In the case of 1H NMR spectrum of the oligomers, obtained via the selective reduction of PHBHx (Fig. 2, Scheme 2), beside the signals corresponding to the protons of 3-hydroxybutyrate and 3-hydroxyhexanoate repeating units, the additional signals that are derived from the protons of PHBHx oligodiols end groups (that contain primary and secondary hydroxyl groups) were also observed. Thus, the signals denoted a, b and c were assigned to the protons of the 3-hydroxybutyrate end group, whereas the signals denoted d, e, f and g were assigned to the protons of the 1-methyl-3-hydroxypropyl end group. Additionally, signals (designated as h–s, Fig. 2, Scheme 2) which represent the protons of 3-hydroxyhexanoate and the 1-propyl-3-hydroxypropyl end groups (which were formed due to the reduction of the 3-hydroxyhexanoate-co-monomer unit) were observed.
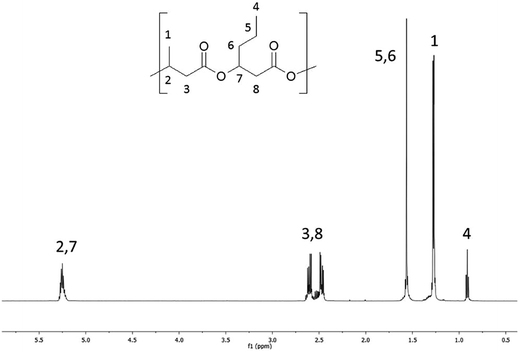 |
| Fig. 1 1H NMR spectrum of starting poly(3-hydroxybutyrate-co-3-hydroxyhexanoate), PHBHx. | |
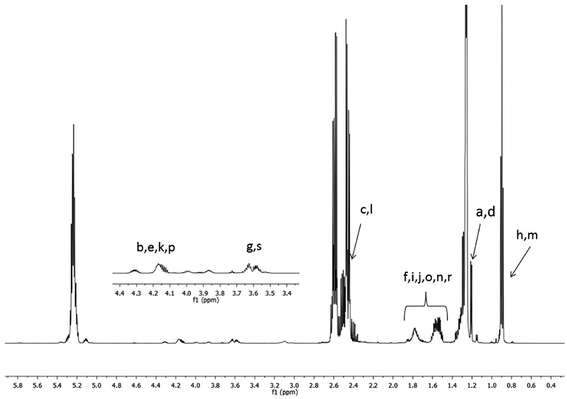 |
| Fig. 2 1H NMR spectrum of oligo(3-hydroxybutyrate-co-3-hydroxyhexanoate) diols obtained via reduction of high molar mass PHBHx biopolyester. Refer to Scheme 2 for the chemical structures of the oligomers. | |
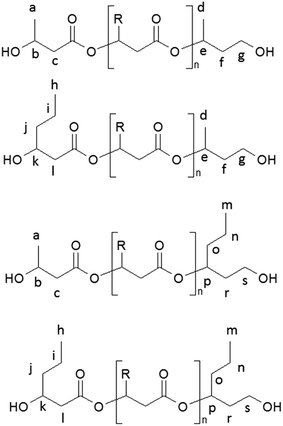 |
| Scheme 2 The chemical structures of oligo(3-hydroxybutyrate-co-3-hydroxyhexanoate) diols obtained via controlled reduction of PHBHx biopolyester. | |
Due to the random distribution of 3-hydroxybutyrate and 3-hydroxyhexanoate co-monomer units in the starting PHBHx biopolyester, the selective reduction of this biopolyester leads to the PHBHx oligodiols differing in the structure of the terminal groups. The chemical structures of the PHBHx oligomers received via controlled reduction of PHBHx are shown in Scheme 2.
Based on the integration of the signals of protons in the 1H NMR spectra (Fig. 1 and 2), the content of 3-hydroxybutyrate and 3-hydroxyhexanoate co-monomer units was estimated. The chemical composition of the oligomers obtained after reduction was close to the composition of the starting PHBHx biopolyester. The content of the 3-hydroxyhexanoate units in the obtained PHBHx oligodiols was estimated in the range from 12 to 12.6 mol%.
However, in the 1H NMR spectrum of oligomers obtained via selective reduction of biopolyester PHBHx (Fig. 2), the assignment of the signals of end groups was not conclusive, because the signals characteristic for the protons of aliphatic group in β-position of 3-hydroxyhexanoate units, as well as the signals of protons of the end groups formed by reduction were overlapped. Therefore, verification of the structure of oligomers obtained by the reduction of PHBHx was carried out using mass spectrometry.
Structural characterisation of the PHA oligodiols by ESI-MS and ESI-MS/MS
Development of soft ionization techniques in mass spectrometry facilitates solving the difficult questions regarding the molecular structure of copolymers.14,29–33 To obtain precise information on the structure of the resulting PHBHx oligodiols at the molecular level, electrospray mass spectrometry ESI-MS was used. This technique is very useful for the structural characterisation of partially degraded PHA biopolyesters, especially for characterization of the chemical structure of the end groups of analysed oligomers.
Fig. 3 shows the ESI-MS spectrum of the oligodiols obtained via selective reduction of PHBHx biopolyester. This spectrum consists of one main series of clusters of singly charged sodium adducts. The ions presented in the spectrum were separated due to their different degrees of oligomerization and composition. The differences between m/z value of the signals belonging to the neighboring clusters are equal to 86, which correspond with the molar mass of the 3-hydroxybutyrate (3-HB) repeating units. The differences between m/z value of ions within the cluster with the same degree of oligomerization are equal to 28, which corresponds with the difference between the molar mass of individual the 3-hydroxyhexanoate (3HH) and 3-hydroxybutyrate (3HB) co-monomer units. The presence in the mass spectrum of PHBH oligodiols of the cluster ions with different degree of oligomerization and composition is better visible in the expanded region of the mass spectrum shown in the Fig. 3b.
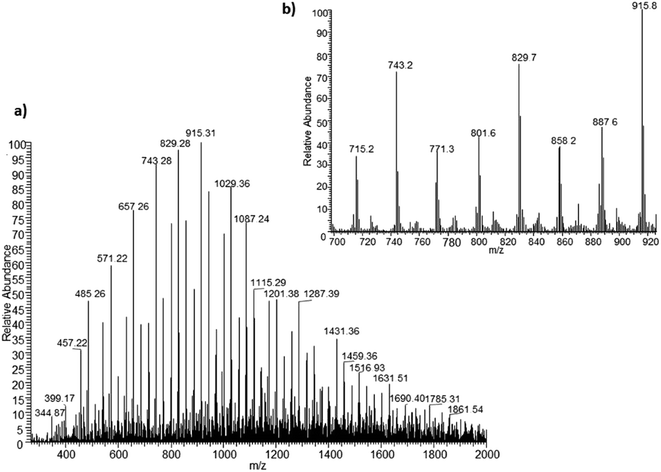 |
| Fig. 3 ESI-MS spectrum (positive-ion mode) of the oligodiols obtained via selective reduction of PHBHx (a). Expansion of basic ESI-MS spectrum in the mass range m/z 700–920 (b). | |
The presence of one main series of cluster ions for the mass spectra of obtained oligodiols clearly demonstrates the high selectivity of the reduction process. However, through the reduction of the PHBHx macromolecules, which contain two statistically distributed 3HB and 3HH co-monomer units, oligodiols with different combinations of end groups (3-hydroxybutyrate, 1-methyl-3-hydroxypropyl, 3-hydroxyhexanoate and 1-propyl-3-hydroxypropyl) were obtained. The general structures of the sodium adducts of oligo(3-hydroxybutyrate-co-3-hydroxyhexanoate)diols present in ESI-MS spectrum were depicted in the Scheme 3. Based on the mass assignment of singly charged ions observed in the mass spectrum, the structures of the end groups and repeating units can be inferred. The structural assignments of ions appearing in the expanded region (m/z 700–920) of the ESI-MS spectrum of PHBHx oligodiols was proposed in Table 2.
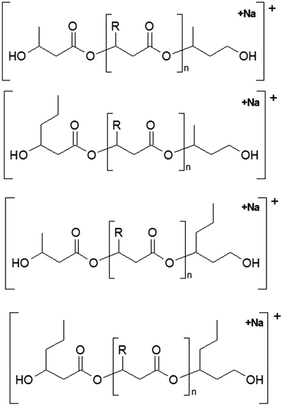 |
| Scheme 3 The chemical structures of the sodium adduct of oligo(3-hydroxybutyrate-co-3-hydroxyhexanoate)diols presented in the ESI-MS spectrum in Fig. 3. | |
Table 2 The structural assignments of one ions cluster appearing in the expanded region (m/z 700–920) of the ESI-MS spectrum of PHBHx oligodiols
m/z |
End group |
Oligomer chain |
End group |
715 |
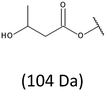 |
–[HB]6– |
 |
801 |
–[HB]7– |
887 |
–[HB]8– |
743 |
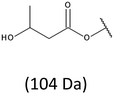 |
–[HB]5[HH]– |
 |
829 |
–[HB]6[HH]– |
915 |
–[HB]7[HH]– |
743 |
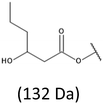 |
–[HB]6– |
 |
829 |
–[HB]7– |
915 |
–[HB]8– |
743 |
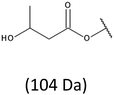 |
–[HB]6– |
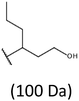 |
829 |
–[HB]7– |
915 |
–[HB]8– |
771 |
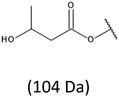 |
–[HB]4[HH]2– |
 |
857 |
–[HB]4[HH]2– |
943 |
–[HB]4[HH]2– |
771 |
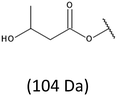 |
–[HB]5[HH]– |
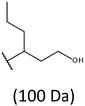 |
857 |
–[HB]6[HH]– |
943 |
–[HB]7[HH]– |
771 |
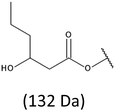 |
–[HB]5[HH]– |
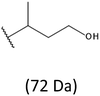 |
857 |
–[HB]6[HH]– |
943 |
–[HB]7[HH]– |
771 |
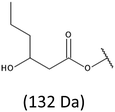 |
–[HB]6– |
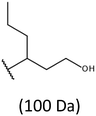 |
857 |
943 |
–[HB]7– |
–[HB]8– |
Thus, the most abundant sodium adduct in this mass spectrum was located at m/z 915, corresponding to the sodiated oligo(3-hydroxybutyrate-co-3-hydroxyhexanoate)diols containing eight 3HB repeating units and one 3HH unit located along the chain or as a group connected with secondary hydroxyl end group or in the reduced form as (1-propyl-3-hydroxypropyl) end group (see Table 2).
On the expanded region of mass spectrum in the mass range m/z 700–920 (Fig. 3b), the additional minor series of ions can be observed. This series with low intensities correspond to the sodium adducts of oligo(3-hydroxybutyrate-co-3-hydroxyhexanoate) that are terminated by 3-hydroxylbutyrate/3-hydroxylhexanoate and 3-hydroxybutyric/3-hydroxyhexanoic acid end group. The presence in the mass spectrum of oligo(3-hydroxybutyrate-co-3-hydroxyhexanoate) terminated by hydroxyl and carboxyl end group is probably due to the presence of such end groups in the chains of pure PHBHx biopolyester. The carboxylic end groups do not undergo reduction under these conditions.
More detailed insight into the structure of individual macromolecular ions of the PHBHx oligodiols was obtained by using tandem ESI-MS/MS spectrometry. Application of this kind of analysis allows to verify the chemical structure of end groups, as well as the molecular structure of oligomers' chain (the results Scheme 1S and Fig. 1S–3S are given in ESI†).
Synthesis of novel terpolyester based on oligo(3-hydroxybutyrate-co-3-hydroxyhexanoate) and sebacic acid
The defined at the molecular level PHBHx oligoesterdiols with different molar masses were then used in the synthesis of new biodegradable polymeric materials via polycondesation reaction with sebacoyl chloride, (Scheme 4).
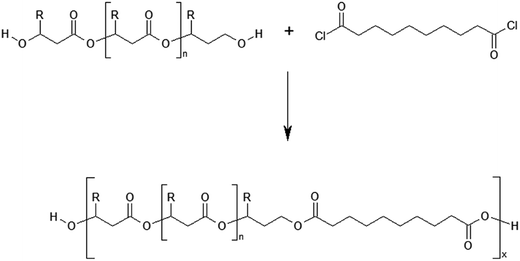 |
| Scheme 4 Polycondensation reaction of PHBHx oligodiols with sebacoyl chloride. R = CH3 for 3HB units or R = C3H7 for 3HH units. | |
The process of polycondensation was performed in bulk, using the equimolar ratio of oligoesterodiols to sebacoyl chloride. It was assumed that the application PHBHx oligodiols with different average molar masses in policondesation reaction, while maintaining an equimolar ratio of the both substrates, should allow to obtain a new polyester with varying content of units derived from sebacic acid. As a result of the polycondensation processes which were conducted, the terpolyesters containing three kinds of constitutional units: 3-hydroxybutyrate (3HB), 3-hydroxyhexanoate (3HH) and constitutional units derived from sebacic acid (SEB) were obtained. The average molecular weight of the obtained terpolyesters determined by GPC technique is summarized in Table 3.
Table 3 Values of average molar mass, dispersity and thermal properties such as Tg, Tm, and Tmax for obtained terpolyestersa
Nr |
Polyester |
Mn |
Mw/Mn |
Tg [°C] |
Tm [°C] |
Tmax [°C] |
PHBHx0 – starting biopolyester, PHBHx1 – biopolyester with similar average molar mass as novel terpolyester. |
0 |
PHBHx0 |
700 000 |
3.5 |
1.0 |
115.0 |
291 |
1 |
PHBHx1 |
10 000 |
1.4 |
−0.8 |
105.0 |
291 |
2 |
Poly(86% 3HB-co-10% 3HH-co-4% SEB) |
7000 |
2.0 |
−11.0 |
99.2 |
291 |
3 |
Poly(82% 3HB-co-12% 3HH-co-6% SEB) |
7000 |
1.6 |
−12.7 |
95.5 |
295 |
4 |
Poly(80% 3HB-co-11% 3HH-co-9% SEB) |
8000 |
1.7 |
−14.9 |
92.7 |
298 |
The structure and chemical composition of obtained terpolyester were determined by NMR technique. Due to the fact that on the 1H NMR spectrum, the signals corresponding to the various protons characteristic for constitutional units displayed the same overlap, which limited a thorough analysis of the spectrum, the chemical structures of the studied polyesters were determined by carbon nuclear magnetic resonance (13C NMR).
The 13C NMR spectrum of terpolyester obtained via polycondensation PHBHx oligoesterodiols and sebacoyl chloride is shown in Fig. 4.
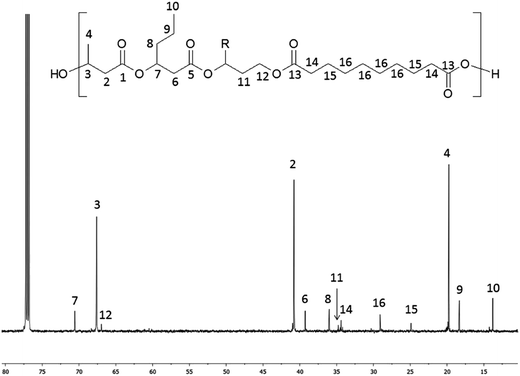 |
| Fig. 4 13C NMR spectrum of obtained terpolyester poly(3HB-co-3HH-co-SEB) (Table 3, terpolyester 2). R = CH3 for 3HB or R = C3H7 for 3HH units. | |
In the 13C NMR spectrum of obtained terpolyester, signals corresponding to the carbon atoms of constitutional units derived from PHBHx and sebacic acid units (SEB) were observed, which are marked as numbers from 1 to 16. The signals characteristic for carbon of 3HB units were designated as 1–4, 3HH units designated as 5–10 and SEB units designated as 13–16. Additionally, the signals (designated as 11–12), characteristic for a carbon of end groups of PHBH oligoesterodiol formed via reduction, were visible in the spectrum. Only these two signals were detected, because signals from other carbons present in reduced HB or HH units overlap with signals from 3HB and 3HH repeated units.
The 13C NMR spectrum of terpoliesters confirmed the presence of three different constitutional units in the polymer chain. Using 13C NMR spectra, the co-monomer composition of obtained terpolyesters was determined. Application in polycondensation of the PHBH oligoesterodiols with different average molar masses allowed to obtain the terpolyester with different chemical composition: poly(86% 3HB-co-10% 3HH-co-4% SEB), poly(82% 3HB-co-12% 3HH-co-6% SEB) and poly(80% 3HB-co-11% 3HH-co-9% SEB).
The thermal properties of obtained terpolyesters were determined by TGA and DSC techniques. The decomposition curves and maximum decomposition temperature rate Tmax of terpolyesters were determined with the aid of TGA technique. Whereas, the DSC technique was used for determination of glass transition temperature Tg and the melting point Tm. The results of the TGA and DSC analysis are summarized in Table 3. For comparison of the thermal properties, the terpolyesters with similar average molar masses were used. Additionally, the properties of the newly obtained materials were compared with the properties of the PHBHx starting biopolyester.
The analysis of Table 3 shows that the obtained terpolyesters have a higher thermal stability than the raw PHBHx biopolyester. Furthermore, the value of the maximum rate of decomposition temperature Tmax increases with the increasing content of SEB constitutional units in the polyester chain. Based on the DSC analysis, with the increase of the content of SEB constitutional unit in the polyester chain, the decrease of the glass transition temperature was observed. The lowest value Tg at −14.9 °C was reached for the terpolyester with the composition of poly(80% 3HB-co-11%3HH-co-9% SEB). Simultaneously with the increase of SEB unit content in the polyester chain, the decrease in melting point temperature Tm was also observed. When comparing the Tg and the Tm values obtained for terpolyesters and output PHBHx biopolyester it is easy to observe a definite decline in the Tg value, while relatively high Tm values are maintained. Such a phenomenon is important for the possibility of further use of terpolyester obtained as construction materials.
The new polyester materials described here have greater thermal stability than the starting biopolyester. Furthermore, the introduction units of sebacic acid to a polyester chain leads to enhanced elasticity of the material and reduces the required processing temperature.
Poly(80% 3HB-co-11% 3HH-co-9% SEB) biopolymer is non-toxic to human cells
To ensure that poly(80% 3HB-co-11% 3HH-co-9% SEB) is not toxic to human cells, coated coverslips were seeded with either human embryonic kidney cells (HEK293s) or adult normal lung fibroblasts (WI-38s). Following 5 days of incubation, the viability of these cells was assessed using a standard MTT assay. For comparison, equal numbers of cells were seeded directly onto tissue culture treated wells of the same size. The MTT assay showed that there was no significant difference in cell viability between cells grown on (optimal) tissue-culture treated plastic or the poly(80% 3HB-co-11% 3HH-co-9% SEB)polymer directly (Fig. 5A). This indicates that poly(80% 3HB-co-11% 3HH-co-9% SEB) polymer conveys no toxicity to human cells in vitro. When observing these cells by phase-contrast microscopy (Fig. 5B) both the HEK293s and WI-38s appear healthy and have their normal characteristic appearance.34,35
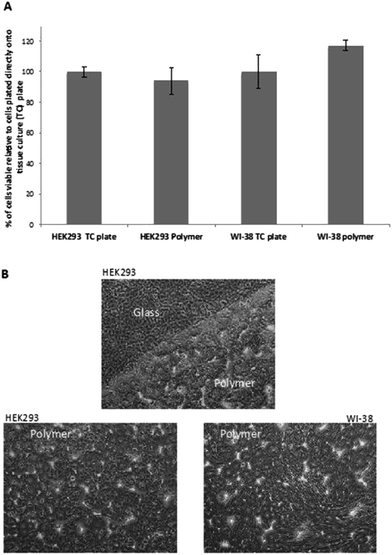 |
| Fig. 5 Poly(80% 3HB-co-11% 3HH-co-9% SEB) polymer in not toxic to human cells. (A) Human embryonic kidney cells (HEK293s) or adult normal lung fibroblasts (WI-38s) grew on polymer coated coverslips with no significant difference in viability compared to cells grown on plastic wells specifically treated to grow mammalian cells on (error bars = SD). (B) Both HEK293s and WI-38s attached to and proliferated on the poly(80% 3HB-co-11% 3HH-co-9% SEB) polymer. The polymer did not completely cover each coverslip, the top panel shows HEK293s growing at the interphase between the edge of the polymer and the glass coverslip beneath it. The bottom two panels show many viable HEK293 (left) and WI-38 (right) cells in the center of the polymer coated coverslip. The appearance of these cells is consistent with that seen when grown under standard conditions (all images 100×). | |
In this study, the poly(3HB-co-3HH-co-SEB) terpolyesters obtained due to their biocompatibility and good thermomechanical properties were used to produce three-dimensional scaffolds for cell cultures. The scaffolds were prepared by the solvent casting particulate leaching method (SCPL) using sodium chloride as a blowing agent.
The preliminary studied performed using poly(3HB-co-3HH-co-SEB) terpolyesters prepared as a three-dimensional spatial structures, presented at Fig. 6, confirmed that it can act as a biocompatible scaffolds for cell cultures. The SEM micrograph of scaffold obtained with the use of novel terpolyesters show that applied salt leaching from mixture of polymers and sodium chloride allows to obtain dense and porous structure suitable for using to cell growth in tissue engineering.
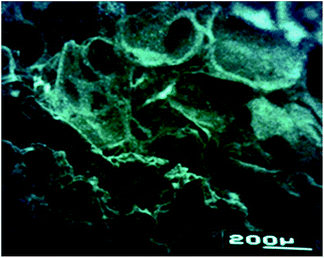 |
| Fig. 6 SEM images of scaffold prepared from terpolyester poly(3HB-co-3HH-co-SEB) by elution. | |
Conclusions
In the present study we have modified the properties of PHA biopolyester, by synthesizing new terpolyesters, comprising structural segments derived from PHA, connected with constitutional units containing a relatively long aliphatic chain originating from sebacic acid, via polycondensation process. According to the current knowledge, it is not possible in the course of the biotechnological process to obtain these kinds of polyesters.
In the synthesis of the novel polyesters, the oligoesterdiols obtained by controlled reduction process of PHBHx biopolyesters and derivatives of sebacic acid were used. The method of controlled reduction of PHA using lithium borohydride (LiBH4), developed by us, allowed to obtain uniform PHBHx oligomers with different average molar masses which were then used in polycondensation process with derivatives of sebacic acid.
The use of PHBHx oligomers with varying molecular masses and sebacoyl chloride in polycondensation reaction with constant molar ratio 1
:
1 allows to obtain novel terpolyesters with a different percentage share of repeating unit from sebacic acid. The analysis carried out with the aid of DSC and TGA shows that presence of repeating unit from sebacic acid leads to decrease of Tg and Tm and an increase of Tmax. Moreover, the decrease of Tg is higher than Tm, what allows to maintain the structural properties, in contrast to the natural polyesters (mcl-PHA), wherein the elongation of side chain in the repeating units simultaneously significantly decreases Tg and Tm, what makes the use of such materials as construction materials impossible.
Additionally, we have shown that the poly(3HB-co-3HH-co-SEB) polymer is not toxic to human cells in vitro. Moreover, phase-contrast microscopy indicates that human cells growing on the polymer appear healthy and have their normal characteristic appearance.
Finally, it should be highlighted that the application of PHA oligoesterodiols in polycondensation process with sebacoyl chloride showed potential in the synthesis of new terpolyesters with more favorable properties for application in regenerative medicine than raw biopolyesters PHA.
Acknowledgements
This work was supported by Polish National Science Centre, contract DEC-2013/11/B/ST5/02222. Biological studies were funded by the Research Investment Fund, University of Wolverhampton School of Biology, Chemistry and Forensic Science, Faculty of Science and Engineering, UK.
References
- G. Braunegg, G. Lefebvre and K. F. Genser, J. Biotechnol., 1998, 65, 127–161 CrossRef CAS PubMed.
- M. Zinn, B. Witholt and T. Egli, Adv. Drug Delivery Rev., 2001, 53, 5–21 CrossRef CAS PubMed.
- J. Asrar and J. C. Hill, J. Appl. Polym. Sci., 2002, 83, 457–483 CrossRef CAS.
- E. Zagar, A. Krzan, G. Adamus and M. Kowalczuk, Biomacromolecules, 2006, 7, 2210–2216 CrossRef CAS PubMed.
- S. F. Williams and D. P. Martin, Polyesters III, applications and commercial products, in Biopolymers, ed. Y. Doi and A. Steinbüchel, Wiley, Weinheim, 2002, vol. 4, p. 91 Search PubMed.
- G. Q. Chen and Q. Wu, Biomaterials, 2005, 26, 6565–6578 CrossRef CAS PubMed.
- V. Piddubnyak, P. Kurcok, A. Matuszowicz, M. Głowala, A. Fiszer-Kierzkowska, Z. Jedliński, M. Juzwa and Z. Krawczyk, Biomaterials, 2004, 25, 5271–5279 CrossRef CAS PubMed.
- G. Adamus, W. Sikorska, H. Janeczek, M. Kwiecień, M. Sobota and M. Kowalczuk, Eur. Polym. J., 2012, 48, 621–631 CrossRef CAS.
- D. Kai and X. J. Loh, ACS Sustainable Chem. Eng., 2014, 2, 106–119 CrossRef CAS.
- D. P. Martin and S. F. Williams, Biochem. Eng. J., 2003, 16, 97–105 CrossRef CAS.
- G. Q. Chen, Chem. Soc. Rev., 2009, 38, 2434–2446 RSC.
- Z. W. Dai, X. H. Zou and G. Q. Chen, Biomaterials, 2009, 30, 3075–3083 CrossRef CAS PubMed.
- X. Y. Xu, X. T. Li, S. W. Peng, J. F. Xiao, C. Liu, G. Fang, K. C. Chen and G. Q. Chen, Biomaterials, 2010, 31, 3967–3975 CrossRef CAS PubMed.
- G. Adamus, W. Sikorska, M. Kowalczuk, I. Noda and M. M. Satkowski, Rapid Commun. Mass Spectrom., 2003, 17, 2260–2266 CrossRef CAS PubMed.
- G. Adamus, Macromol. Symp., 2006, 239, 77–83 CrossRef CAS.
- G. Adamus, W. Sikorska, M. Kowalczuk, M. Montaudo and M. Scandola, Macromolecules, 2000, 33, 5797–5802 CrossRef CAS.
- S. Nguyen, G. Yu and R. H. Marchessault, Biomacromolecules, 2002, 3, 219–224 CrossRef CAS PubMed.
- M. Kawalec, G. Adamus, P. Kurcok, M. Kowalczuk, I. Fortran, M. L. Focarete and M. Scandola, Biomacromolecules, 2007, 8, 1053–1058 CrossRef CAS PubMed.
- M. Kawalec, M. Sobota, M. Scandola, M. Kowalczuk and P. Kurcok, J. Polym. Sci., Part A: Polym. Chem., 2010, 48, 5490–5497 CrossRef CAS.
- M. Kwiecień, M. Kawalec, P. Kurcok, M. Kowalczuk and G. Adamus, Polym. Degrad. Stab., 2014, 110, 71–79 CrossRef.
- A. Ballistreri, D. Garozzo, M. Giuffrida, G. Impalloment and G. Montaudo, Macromolecules, 1989, 22, 2107–2111 CrossRef CAS.
- R. Lehrle, R. Williams, Ch. French and T. Hammond, Macromolecules, 1995, 28, 4408–4414 CrossRef CAS.
- Z. Spitalsky, I. Lacik, E. Lathova, I. Janigova and I. Chodak, Polym. Degrad. Stab., 2006, 91, 856–861 CrossRef CAS.
- M. Kwiecień, G. Adamus and M. Kowalczuk, Biomacromolecules, 2013, 14, 1181–1188 CrossRef PubMed.
- Y. Wang, G. A. Ameer, B. J. Sheppard and R. Langer, Nat. Biotechnol., 2002, 20, 602–606 CrossRef CAS PubMed.
- G. Liu, B. Hinch and A. D. Beavis, J. Biol. Chem., 1996, 271, 25338–25344 CrossRef CAS PubMed.
- A. V. Grego and G. Mingrone, Clin. Nutr., 1995, 14, 143–148 CrossRef CAS PubMed.
- Z. You, H. Cao, J. Gao, P. H. Shin, B. W. Day and Y. Wang, Biomaterials, 2010, 31, 3129–3138 CrossRef CAS PubMed.
- G. Adamus, Macromolecules, 2009, 42, 4547–4557 CrossRef CAS.
- I. Kwiecień, T. Bałakier, J. Jurczak, M. Kowalczuk and G. Adamus, Rapid Commun. Mass Spectrom., 2015, 29, 533–544 CrossRef PubMed.
- G. Adamus, I. Kwiecień, M. Maksymiak, T. Bałakier, J. Jurczak and M. Kowalczuk, Anal. Chim. Acta, 2014, 808, 104–114 CrossRef CAS PubMed.
- M. Maksymiak, T. Bałakier, J. Jurczak, M. Kowalczuk and G. Adamus, RSC Adv., 2016, 6, 57751–57761 RSC.
- M. Michalak, M. Kwiecień, M. Kawalec and P. Kurcok, RSC Adv., 2016, 6, 12809–12818 RSC.
- H. Liebhaber, S. Krugman, J. P. Giles and D. M. McGregor, Virology, 1964, 24, 109–113 CrossRef CAS PubMed.
- N. L. Simmons, Exp. Physiol., 1990, 75, 309–319 CrossRef CAS PubMed.
Footnote |
† Electronic supplementary information (ESI) available. See DOI: 10.1039/c6ra27980e |
|
This journal is © The Royal Society of Chemistry 2017 |
Click here to see how this site uses Cookies. View our privacy policy here.